Improved performance and lifetime of lithiumion batteries is important for automobile manufacturers that produce cars powered electrochemically by lithium-ion battery packs. Electrochemical measurements are commonly used to correlate capacity loss and impedance increase to the formation of degradation products in the solid electrolyte interface (SEI). Chemical analysis can complement electrochemical measurements by identifying degradation products.
Ion chromatography (IC) combined with high-resolution accurate-mass spectrometry (HRAMS) effectively characterizes ionized and ionizable components in electrode samples from aged lithium-ion batteries. IC-HRAMS is used to identify probable chemical species in the analytes based on both ion-exchange selectivity behavior and on exact mass to an accuracy of at least four decimal places.
This article focuses on the use of gradient anion-exchange ion chromatography with a conductivity detector coupled with HRAMS with an electrospray interface (ESI) to analyze the degradation products obtained from surface deposits on lithium-ion battery anodes, which are cycle- and calendar-aged to varying degrees of capacity loss. Information on the anion-exchange elution behavior correlates with chemical-formula information from HRAMS to provide accurate results. Compound classes and specific compounds found in these anode wash samples include solvent-degradation products such as methyl carbonate; anionic contaminants such as chloride and sulfate; electrolyte breakdown products such as fluoride, phosphate and pyrophosphate; organic acids derived from degradation of the anode; as well as ionic materials generated from reactions between various ion classes found in the samples, including sulfate esters, phosphate esters and fluorophosphate esters. Using IC on samples containing complex mixtures of anionic species can help elucidate chemical structures of unknown components.
HRAMS provides accurate analyte identification, while IC delivers confirmatory information about ionizable functional groups. Together, these techniques provide insight into the identity of anionic degradation products in lithium-ion batteries.
Figure 1 – Ion chromatograph with conductivity detector and HRAMS.Experimental
Figure 1 is a schematic of the ion chromatograph with conductivity detector and HRAMS (IC-CD-HRAMS) hardware. Thermo Scientific Xcalibur and Thermo Scientific Dionex Chromeleon Chromatography Data System (CDS) software were used for system control and data collection.
Ion chromatography
Sample components were separated by anion-exchange selectivity. Instrumentation was as follows: 1) Thermo Scientific ICS2100 Reagent-Free Ion Chromatograph (Sunnyvale, Calif.)—pump, eluent generator fitted with a KOH EGC cartridge and a conductivity detector; 2) Thermo Scientific Dionex AXP pump—delivered 90/10 v/v acetonitrile/deionized water to a mixing tee positioned after the conductivity detector cell and before the electrospray inlet to the mass spectrometer—gradient elution conditions are shown in Table 1; 3) Thermo Scientific IonPac AS11 (250 × 2-mm i.d.) analytical separation column; and 4) Thermo Scientific Dionex AERS 500 (2-mm) suppressor.
Table 1 – HRAMS data generated using Q Exactive Orbitrap mass spectrometer with electrospray interface operating in negative mode
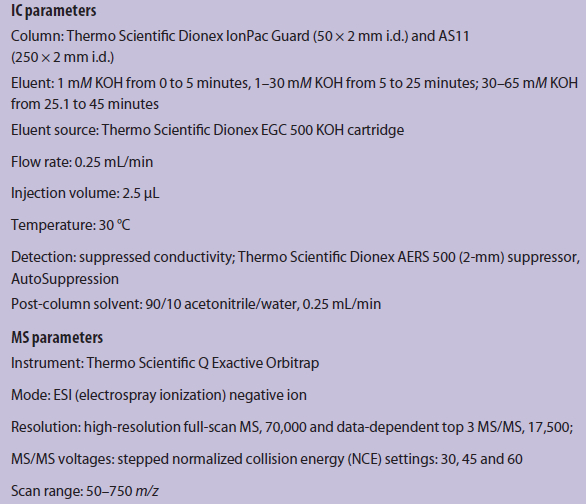
High-resolution accurate-mass spectrometry
HRAMS data was generated using a Thermo Scientific Q Exactive Orbitrap mass spectrometer with an electrospray interface operating in the negative mode.1 (see Table 1).
Sample preparation
Carbon-based anodes extracted from battery cells aged in different ways were washed with deionized water; the wash solutions were filtered prior to injection into the IC-HRAMS system. The battery cells contained a mixed organic-carbonates electrolyte with lithium hexafluorophosphate. The objectives of the sample preparation were to screen samples to identify compounds and determine any difference among the samples with various aging histories, and to use the features of the anion-exchange separation to help identify analyte properties.
The anode samples, obtained from an automobile manufacturer, included a control non-aged sample, a 45 °C calendar-aged sample exhibiting 20% loss in capacity and two cycle-aged samples from accelerated operation at 35 °C showing 30% loss and 40% loss in capacity. Process blanks were also included.
Surface deposits were observed on the anodes. All of the anode samples were cut to a known weight, then sonicated and rinsed in weighed amounts of water. The extracts were filtered using Whatman PP 0.45-μm filters (GE Healthcare Life Sciences, Pittsburgh, Penn.), and the weight losses were calculated. The filtered extracts were injected into the IC-CD-HRAMS system.
Results and discussion
Valency in IC and ESI
Anions were separated based on anion-exchange selectivity relative to hydroxide using gradient-elution conditions.2 The sample cations and potassium from the eluent were removed using an inline electrolytic suppressor to replace these cations with hydronium ion, which was simultaneously neutralized to water with the eluent hydroxide ion, leaving a low-conductance background for detection of the sample anions by electrical conductivity. This process also desalted the column effluent before it was mixed with acetonitrile and entered the electrospray interface of the mass spectrometer. It is worth noting that anions are separated on the anion-exchange column according to their valency in the high-pH environment of the eluent, but after traveling through the suppressor they usually enter the mass spectrometer with one charge. Therefore, species identified in the mass spectrometer bear a –1 charge. For example, sulfate is separated as a divalent anion, but is detected in the mass spectrometer as hydrogen sulfate.
Ions confirmed on degradation pathways
Vortmann3 proposed a degradation pathway that included hydrolysis of lithium hexafluorophosphate followed by esterification of several hydrolysis and decomposition products, some requiring small amounts of water as a reactant. The current authors have been able to confirm the presence of many of the phosphorus-containing species shown in the Vortmann pathway, including methyl hydrogen phosphate, dimethyl phosphate, monofluorophosphate methyl ester and phosphate, among others. Understanding a degradation process and which pathway to block to prevent the formation of key breakdown products can inhibit degradation and lead to safer, longer lasting lithium-ion batteries.
Valency and peak identification
Figure 2a and b show the elution of anions detected by the conductivity detector (a) and by the mass spectrometer (b). The detectors are complementary in that small ions such as fluoride (retention time 3.7 minutes) are best detected in the conductivity trace, while ions with higher mass are detected and fragmented for chemical identification by the mass spectrometer. Since the elution was accomplished using an increasing concentration gradient of hydroxide, the analytes generally elute in this order: monovalent, divalent, trivalent and higher. Thus, retention time relative to known analytes such as monovalent chloride, divalent sulfate and trivalent phosphate provides information on the ionizable functional groups present in the unknowns that elute in those regions of the chromatogram. This information is especially useful when combined with the accurate-mass data to identify possible chemical composition.
Figure 2 – Elution of anions detected by the conductivity detector (a) and the mass spectrometer (b).Table 2 – Peak identifications consistent with both anion exchange and mass spectrometry data
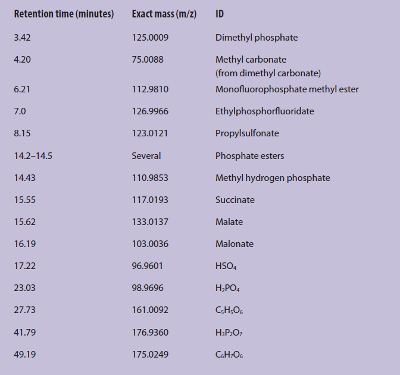
Structural information based on ion-exchange selectivity can be used with that from the HRAMS to give a more complete picture of the structure of the unknowns (Figure 3). The retention time window in the anion-exchange gradient-elution chromatogram for trivalent species is labeled as such. The peak at 27.73 minutes elutes in the trivalent elution region, and the mass spectral information is shown. The accurate mass of 161.0092 m/z for a possible chemical formula of C5H5O6 has a mass accuracy of 0.2 ppm. In general, mass accuracy values less than 2 ppm are considered high-confidence matches. The ion-exchange properties suggest a tricarboxylate structure, so a possible chemical identification is ethanetricarboxylate. Identification of a compound of interest with four-decimal-point accuracy is extremely important, since, in addition to validating a proposed degradation pathway, it avoids the need for additional resources. The possible chemical identification based on both the behavior by anion exchange and four-decimal-place accuracy of mass-to-charge in the HRAMS is shown in Table 2.
Figure 3 – a) Elution of anions from a cycled sample exhibiting 40% capacity loss. b) HRAMS spectrum for peak at 27.73 min. Conditions in Table 1.Conclusion
Ion chromatography coupled with high-resolution accurate-mass spectrometry helped elucidate the degradation pathways of lithium-ion batteries. Information on the valency of functional groups present in each analyte was instrumental in defining the structural properties of degradation products obtained from surface deposits on lithium-ion battery anodes with varying degrees of capacity loss. Compound classes and specific compounds found in these samples are listed above.
Results from this study suggest that identifying key electrolyte degradation products using chemical analysis may complement electrochemical measurements in areas such as charge transfer and failure analysis testing. In addition to determining correlation of a relative change in battery performance with formation of a specific degradation product (or class of products), information on chemical identification can provide insight into mechanistic pathways. Actions can then be taken to block the formation of a degradation product known to inhibit battery performance and provide improved degradation resistance, leading to longer-lasting lithium-ion batteries.
References
- Zubarev, R.A. and Makarov, A. Orbitrap mass spectrometry. Anal. Chem. 2013, 85, 5288–96.
- Weiss, J. Ion Chromatography, 2nd ed., VCH: Weinheim, Germany, 1995, pp. 360–7.
- Vortmann, B.; Nowak, S. et al. Rapid characterization of lithium ion battery electrolytes and thermal aging products by low temperature plasma ambient ionization high-resolution mass spectrometry. Anal. Chem. 2013, 85(6), 3433–8.
Rosanne W. Slingsby is a senior principal research scientist in the R&D department for Dionex products at Thermo Fisher Scientific, 1228 Titan Way, Sunnyvale, Calif. 94086, U.S.A.; tel.: 408-481-4542; e-mail: [email protected]; www.thermofisher.com. Paul J. Voelker is vertical marketing manager for environmental and industrial markets at Thermo Fisher Scientific. Christopher Pohl is vice president for consumables R&D at Thermo Fisher Scientific. Charanjit Saini is research scientist for Dionex products at Thermo Fisher Scientific. Kate Comstock is senior marketing specialist at Thermo Fisher Scientific. John Moote is a senior chemist at General Motors, Warren, Mich. Michael Balogh is a technical fellow, and Ramona Ying is a staff researcher at General Motors R&D Center, Warren, Mich.