The region of the electromagnetic
spectrum known as terahertz (tera =
1012) has remained relatively unexplored
due to the limitations of
microwave and infrared sources.1–3
Microwave sources, which rely on
electronic propagation of single- or
low-order-guided waves, are only
suitable below 100 gigahertz (giga
= 109), while optically generated IR
multimode beams such as CO2 lasers
are unsatisfactory below 30 THz.
However, new methods for the generation
and detection of THz radiation
have been revealed, including
photoconductive
antennae, electrooptic
crystals, backward-wave oscillators,
and optical mixing.4–6 These
methods have been employed to
generate and detect the frequencies
from 0.1 to over 10 THz, ideal for
detection of rotational and vibrational
energy transitions in polar
molecules such as water.
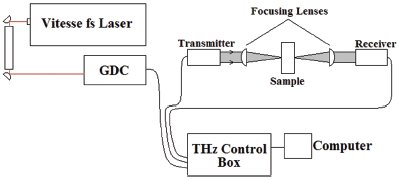
Figure 1 - Basic schematic of THz instrument design.
The commercially available terahertz time–domain spectrometer (THz–TDS) used in this study is shown
schematically in Figure 1 (T-Ray
2000, Picometrix, an Advanced Photonix
company, Ann Arbor, MI). A
Vitesse diode-pumped, mode-locked
Ti:sapphire laser produces <100-fsec
pulses centered at 800 nm. These
pulses are coupled into fiber optic
lines to remove additional steering
optics and provide ease in directing
the beam. To correct for optical pulse
dispersion by the fiber optic components
of the instrument, a grating
dispersion compensator imparts
negative group velocity dispersion
to the optical pulse. Since the pulse
will naturally disperse while traveling
in the fiber optics, it is essentially
compressed negatively to balance
the broadening. The laser pulse
then passes through a beamsplitter,
which directs one resultant beam to
the THz transmitter and the other to
the detector. Both transmitter and
detector consist of photoconductive
antennae, namely low-temperature
grown (LT)-GaAs. When illuminated
by the excitation beam, the
transient current in the biased GaAs
emitter generates THz frequencies.
From the transmitter, the THz radiation
is focused on the sample via a
high-density
polyethylene
(HDPE)
collimating lens, and the radiation
transmitted through the sample
passes through an identical HDPE
lens and on to the detector. The
computer controls a delay line, which
varies the optical delay; this delay
gates the interrogation of the THz
pulse by the initial fsec laser
pulse. Only when the THz
pulse and laser are present on
the antenna simultaneously is
there a current produced; this
current, as a function of delay
time, is the time-dependent
signal. Through Fourier transform (FT) analysis, the time-domain
data can be expressed
in the frequency domain, and
absorbance peaks are evident.
Radiation absorption by water
has been widely explored using
THz systems.7–10 The sheer
number of rotational and vibrational
energy transitions available
in H2O molecules results in broad
absorbance peaks in the THz spectrum
of liquid water, particularly in
the frequency range of 0.3–2.0 THz.
This phenomenon creates potential
for analyzing water-containing samples
having little or no absorptive
characteristics (besides the water
within). One particular system of
interest is aqueous foams.
Foams are used in various applications,
including oil recovery, brewing,
personal care, medicine, and
biotechnology.11,12 Structural properties of the foam in each application
are critical to proper performance
and stability. Due to the fragile and
dynamic nature of foams, these properties
and flow dynamics are difficult
to measure. Temperature fluctuations
and mechanical stresses may cause
foams to degrade or lose functionality.12 Therefore, it is imperative
to have an effective measurement
and qualifying method that characterizes
the foam, yielding drainage
rate data. Foam properties such as
rigidity, structure, and mechanical
strength can be extracted from
drainage rate profiles.13,14
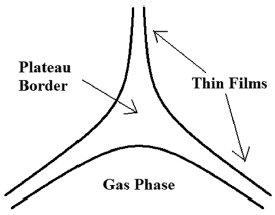
Figure 2 - Interfaces present during drainage of water through foam structures.
Drainage is defined as the liquid
flow between fragile film membranes
(lamellae) via plateau borders
under the influence of gravity
and capillary forces.11 As the
water drains from these lamellar
regions into plateau borders,
the foam gas bubbles become less
stable and are increasingly susceptible
to bursting. The Gibbs-Marangoni stabilization, however,
plays a role in maintaining
bubble life. Here, surfactant molecules
diffuse to thinned areas,
providing additional support before
the bubble bursts. This stabilization
makes it difficult to predict foam
behaviors using models and theory.
Figure 2 labels interfaces of a draining
region consisting of the thin
films (lamellae), a plateau border,
and gas regions.
Several methods currently exist to
study foams. Table 1 lists a fraction
of these methods and touches on the
positives and negatives inherent to
each method.
Table 1 - List of methods used to characterize foams and brief advantages/disadvantages
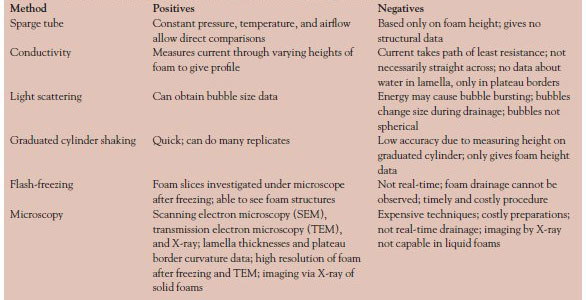
Although the techniques listed offer
important information, many are too
costly, do not provide sufficient data,
or are not feasible for fast-throughput
environments. Also, it is during real-time
drainage that important data are
extracted. Therefore, a method that
can collect real-time data of a draining
foam system is required to sufficiently
characterize the foam properties.
Foam studies have been made possible
using the absorption of THz radiation
by water in aqueous foams. Data have
been obtained on the following systems:
sodium dodecyl sulfate (SDS),
cetyl trimethylammonium bromide
(CTAB), Triton X-114 (Rohm and
Haas, Philadelphia, PA), Ultra Dawn®
dish soap (Procter and Gamble Co.,
Cincinnati, OH), and Guinness beer
(Guinness and Co., Dublin, Ireland).
These samples are representative of
anionic surfactant, cationic surfactant,
nonionic surfactant, mixed surfactants,
and protein surfactant solutions,
respectively. Two different pore sizes
were used with all the systems except the Guinness beer, and five scans were averaged for each system studied.