In recent years, there has been a strong movement toward the use of HPLC columns with smaller particles. While columns with 5-μm particles have long been the standard, some users now have turned to columns of 3.5 μm1 or smaller2 to produce faster separations and increase sample throughput. The advantages of smaller particles are well known: faster separations without sacrificing resolution so that more samples can be analyzed in the same time period. Of course, there are always tradeoffs that must be made to gain this speed. Smaller particles decrease the permeability of columns; thus increased pressures must be used, resulting in increased stress on pumping systems and other instrument components. In addition, columns with particles of ≤3 μm must usually be fitted with end frits of 0.5-μm or 1.0-μm porosity to ensure that smaller particles in the particle distribution do not escape to cause detection and column life problems. These smaller-porosity frits are subject to fouling by microparticulates from samples and mobile phases, eventually causing column failure because of very high backpressures. To eliminate this problem, filtering samples and mobile phases is often a chore that small-particle users must endure.
This article describes a 2.7-μm fused core particle (Advanced Materials Technology, Inc., Wilmington, DE) that has been designed to allow very fast separations without some of the disadvantages of conventional columns with small, totally porous particles. The characteristics of these fused core particles represent a fortunate compromise of separation speed with modest operating pressures. In addition, however, because of the unique structure of a thin porous shell surrounding a uniform solid silica core, columns of these materials exhibit unusually high efficiency. While traditional commercial columns of totally porous particles rarely show reduced plate heights, h (plate height/particle diameter) of less than 2, columns of the fused core particles often exhibit h values of about 1.5 for molecules with molecular weights up to at least 300. This unusually high efficiency is believed by the authors to be a function of the extremely narrow particle size distribution and the higher density of the fused core particles. The end result is a column that produces very fast separations for high sample throughput with conventional equipment and user-friendly operating techniques. The thin outer shell of the particles permits very rapid solute mass transfer (optimized kinetics) so that high mobile phase velocities can be used for fast separations without a significant loss in column efficiency.
Materials and methods
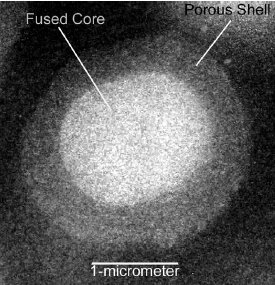
Figure 1 - Electron micrograph cross-section of 2.7-μm fused core particle.
The fused core particles were synthesized in the authors’ laboratory using proprietary nanoparticle technology.3 The highly purified Type B silica particles are 2.7 μm in total diameter with a 0.5-μm-thick outer shell of 90-Å pores, resulting in a nitrogen surface area of ≅150 m2/g. The fused core particles have been given the trademarked name of Halo™, which suggests their physical configuration with the spherical porous shell covering a solid core (Halo fused core columns available from Mac-Mod Analytical, Chadds Ford, PA). A cross-section electron micrograph of one of the particles is shown in Figure 1. Fused core Halo particles are synthesized with an extremely narrow particle size distribution, as indicated in Figure 2.
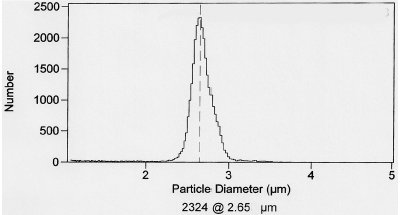
Figure 2 - Particle size distribution of 2.7-μm fused core particles.
Densely bonded monofunctional dimethyl-C8 and dimethyl-C18 stationary phases were prepared on the fused core particles by conventional reactions4 with silanes obtained from Gelest, Inc. (Morrisville, PA). These bonded phase materials were subsequently endcapped with trimethylsilane groups. Carbon/hydrogen analyses were by Micro-Analysis, Inc. (Wilmington, DE). The level of bonded stationary phase was similar to that for totally porous particles and in keeping with that expected for densely bonded materials, where additional silane groups cannot be further reacted because of steric limitations. For example, the bonded phase level on one sample of Halo C18 was 3.29 μeq/m2 prior to endcapping. Particle size measurements were conducted on a Gemini V instrument (Micromeritics, Norcross, GA) and electron microphotographs were obtained from Micron, Inc. (Wilmington, DE). Surface areas were conducted with a Multisizer 3 Coulter Counter (Beckman Coulter, Fullerton, CA).
Chromatographic data were obtained on a model 1100 instrument (Agilent Technologies, Palo Alto, CA) or on a model SPD-6A liquid chromatograph (Shimadzu Scientific Instruments, Tokyo, Japan) using UV detection with a 2-μL cell. Data were recorded from the model 1100 ChemStation (Agilent) or from an in-house-designed computer system using a model 900 interface (PE Nelson, Cupertino, CA). All data were acquired with a detector response time of 0.1 sec, in addition to a data sampling rate of at least 20 points/sec so that a minimum of 20 points would be obtained on the very sharp, low-volume peaks obtained with the fused core Halo columns. Stainless steel column hardware and column frits with 2-μm porosity were from Isolation Technologies, Inc. (Hopedale, MA). The 4.6 × 50 mm and 2.1 × 50 mm columns used in this study were prepared by slurry packing techniques using in-house-designed hardware.5 Chromatographic tests were performed using 1-μL injections from a model 8125 sampling valve (Rheodyne, Cotati, CA). The bed stability study was performed with the model 1100 instrument using a model 1100 series automatic sampler (Agilent Technologies). The 5-μm particle Ace column was from Mac-Mod Analytical, the 3.5-μm particle Zorbax XDB-C18 column was from Agilent Technologies (Wilmington, DE), and the 2.5-μm XBridge C18 column was from Waters Corp. (Milford, MA). Plate heights were calculated with the data systems using the peak half-height/width method.6 Data for the reduced plate height versus mobile phase velocity plots in this paper were fitted to the Knox equation:
where h is the reduced plate height (plate height h/particle size dp); A, B, and C are column coefficients; and u is mobile phase velocity.6
Solvents for mobile phases were from Mallinckrodt Baker (Phillipsburg, NJ). Test solutes were from Sigma-Aldrich (St. Louis, MO). Lorazepam and vantin were obtained by dissolving single commercial pills in the appropriate mobile phase. No effect from inactive excipients in these pills was observed in the authors’ studies.
Results and discussion
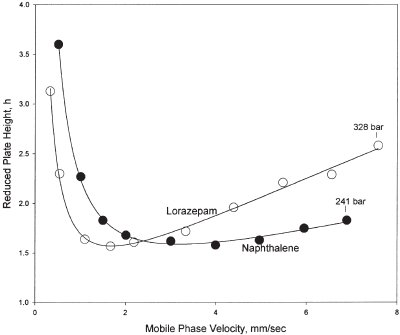
Figure 3 - Comparison of reduced plate height versus mobile phase velocity plots for naphthalene and lorazepam. Column: 50 × 4.6 mm, Halo C8, 2.7 μm. Solutes: naphthalene (MW = 128.2), mobile phase: 60% acetonitrile/40% water; lorazepam (MW = 321.2), mobile phase: 30% acetonitrile/70% 20 mM sodium phosphate buffer, pH 3.5. Temperature: 24 °C.
The unusually high efficiency of fused core Halo columns is illustrated by the data in Figure 3. Here, the reduced plate height minimum for the small molecule, naphthalene (MW 128.2), is about 1.5, representing about 12,000 theoretical plates for this column. Very little increase in plate height occurs for this small molecule when the mobile phase velocity is more than doubled. This effect is the result of the favorable kinetics of the thin porous shell on the fused core Halo particles. The time for solute diffusion in and out of the thin porous shell containing the stationary phase is minimized so that band broadening is also minimized as the mobile phase is increased. Note also in Figure 3 that the reduced plate height for the drug lorazepam (MW 321.2) at the plate height minimum is also about h ~1.5 for column efficiency equivalent to that for the smaller molecule, naphthalene. As the mobile phase velocity is increased for lorazepam, the plate height increases slowly as mobile phase velocity increases as a result of the slower diffusion of the much larger molecule. Yet, at the highest mobile phase velocity (almost 8 mm/sec at 328 bar), lorazepam shows a reduced plate height of about 2.5, indicating that the 50-mm column is still operating with about 7000 theoretical plates. It is believed that the unusually high efficiency of fused core Halo columns is the result of the extremely narrow particle size distribution and the 30–50% higher density for the fused core particles (relative to totally porous particles). These properties apparently allow the formation of a more homogeneous packed bed, which results in efficiencies higher than for conventional totally porous packings (reduced A term, Eq. [1]). While reduced plate heights of 2 for columns is generally accepted as a practical limit, the possibility of increased efficiency for packed HPLC columns as a result of optimized particles was predicted by Knox.7 In the Knox study, reduced plate heights of less than 2 (some as low as 0.6) were obtained with large, solid glass beads and superficially porous particles. The higher density and narrow particle size distribution of the fused core particles appear to be the characteristics that allow reduced plate heights of less than 2 to be a common feature.