Scientists have long needed to view structures and processes that cannot be resolved via traditional optical microscopy. Indeed, much of what is imaged in today’s life science research laboratories is fast-changing cellular activity hidden deep within specimens, making such phenomena especially difficult to resolve.
Ernst Abbe’s work on diffraction-limited optics (published in 1873) revealed that the size of the smallest resolvable feature that can be imaged by an optical microscope is inversely proportional to the light-gathering ability of its objective and proportional to the wavelength of observed light. Light with wavelength λ traveling in a medium with refractive index n and converging to a spot with angle θ will make a spot with radius:
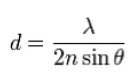
The denominator of the equation refers to the light-gathering potential, or numerical aperture (2× NA), of the optics. In practical terms, the Abbe limit is often assumed to be around d = λ/2.8. Imaging with visible light through typical objectives with an NA of 1 yields an Abbe limit of about 250 nm, which is not sufficient to support observation of some complex intracellular structures and live cellular dynamics. Many fluorescence microscopy techniques, including confocal imaging, multiphoton microscopy and other methods designed specifically to address the challenge of resolution, often require compromises in cost, complexity and sample requirements.
Researchers have numerous options for addressing their need for resolution beyond the diffraction limit of traditional optical microscopy systems. However, most life science researchers cannot jump to high-resolution techniques that abandon light as a means of imaging. These methodologies, such as electron microscopy and atomic force microscopy, either are not capable of sensing the phenomena under study or simply cannot maintain a living specimen through sample preparation and observation. Below are some more practical options being selected in laboratories today.
Imaging at the coverslip
The deeper within the sample one images, the more the limits of diffraction and scatter become a hindrance. Imaging in the near field is one solution for enhancing the resolving power of an optical system. Techniques such as total internal reflection fluorescence (TIRF) microscopy use the evanescent wave that occurs when excitation light passes through a high-refractive index (RI) medium and strikes a lower-RI medium at a critical angle of incidence. At this angle, light is internally reflected before it enters the second medium and produces a strong lateral evanescent electromagnetic field in the lower-RI medium containing the sample, which in turn causes local fluorescent excitation. The result is a very thin (~100 nm) band of excitation at the coverslip/specimen interface. Unwanted background and out-of-focus signals are dramatically reduced with TIRF microscopy, enhancing the researcher’s ability to view very dim and fast-changing events. Though limited to observation at the coverslip, TIRF microscopy provides excellent high-contrast imaging of membrane dynamics and single-molecule events, and continues to be a valuable tool in research.
Super-resolution techniques
In 2014, the Nobel Prize in Chemistry was awarded to researchers who developed various super-resolution microscopy techniques using novel chemistries, hardware and software to accurately determine the location and activity of individual molecules and structures without direct visual observation. Some of the most commonly used super-resolution techniques are STED, PALM, STORM and SIM (Table 1).
Table 1 – Comparison of various types of super-resolution imaging
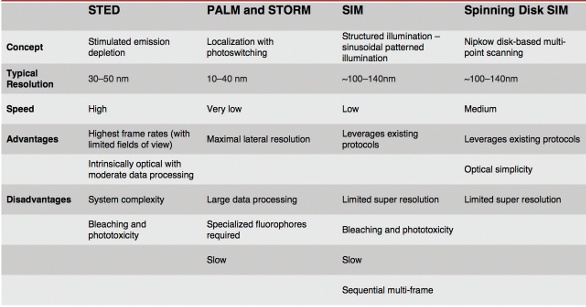
STED
Stimulated emission depletion (STED) microscopy is a powerful deterministic technique that allows images to be captured at resolutions well below the theoretical diffraction limit. In STED, a high-intensity pulsed laser is used to excite the sample. Immediately thereafter, a doughnut-shaped, red-shifted pulse follows. Excited fluorophores exposed to the second STED beam are instantly returned to their ground state by stimulated emission and do not fluoresce, allowing the collection of image data from the remaining excited fluorophores that reside in a small subresolution region at the center of the depleted area. STED’s complexity is its greatest challenge, making it relatively expensive and potentially unavailable to many researchers. In addition, the excitation pulse can damage some samples. Finally, collecting image data can be time-consuming when scanning a large field of view. However, with small fields of view, STED is quite fast.
PALM and STORM
In contrast to STED, which is deterministic, several other techniques rely on localization of fluorescent structures within the sample. Stochastic optical reconstruction microscopy (STORM) and photoactivated localization microscopy (PALM) use fluorescent probes that switch between fluorescent and dark states. Fluorochrome positions can be determined with high precision based on calculating the center positions of each of the fluorescent spots. Through multiple image captures, with each image capturing random subsets of the fluorophores, a super-resolution image is reconstructed from the accumulated position data. Localization methods such as PALM and STORM offer high spatial resolution (20–50 nm), but have low temporal resolutions ranging from 30 seconds to 30 minutes, depending on the application, sample and fluorophores used.
SIM
Structured illumination microscopy (SIM) involves excitation with repeating patterns that interact with the structure of the sample to produce moiré patterns that are used to extrapolate images at higher resolution than would be achievable through traditional unstructured widefield illumination. SIM provides twice the resolution potential of comparable widefield illumination systems and, depending on the implementation, imaging can be performed at relatively high frame rates, making SIM a useful technique for imaging fast-changing events within living samples. In most practical applications, resolution ranges from 100 to 140 nm.
Point-scanning SIM
Most super-resolution techniques rely on software to process image data and provide accurate estimates of the location of subdiffraction-limit structures and dynamics. For mathematical simplicity, most current implementations of SIM use sinusoidal illumination patterns and computational image reconstruction.
However, systems that perform optically what otherwise would be done computationally have advantages in speed and simplicity. Mats Gustafsson, the inventor of SIM technology, asserted that the optical microscope could produce better images at far-higher resolutions by applying structured or patterned excitation. Today, new point-scanning and spinning-disk systems combined with modern detectors, improved optics and insightful mathematical models make super resolution more accessible than ever, just as Dr. Gustafsson predicted.
The spinning disk confocal microscope, first popularized for its reliability and speed in live-cell imaging, can be adapted for super-resolution performance. For instance, the Olympus SD-OSR (Olympus America, Waltham, MA) pairs a high-speed spinning-disk microscope system with a high-performance silicone oil objective for an ideal optical match to living samples , to deliver image resolution up to twice that of a widefield microscope without localization (Figure 1). Resolution-limited excitation, reduced-noise image acquisition and high-performance optics combine with SIM image formation to deliver 110–130 nm resolution at up to three frames per second (Figure 2).
Figure 1 – Comparison of imaging at the diffraction limit vs using super-resolution microscopy. Both (a) and (b) show the same growth cone NG-108, with F-actin in green and tubulin in red. (a) was captured using confocal microscopy; (b) was captured using super-resolution microscopy. (Images courtesy of Kaoru Kato, National Institute of Advanced Industrial Science and Technology [AIST], Biomedical Research Institute, University of Tsukuba, Graduate School of Life and Environmental Sciences, Japan.)
Figure 2 – Stress fibers in a HeLa cell, depicting F-actin (phalloidin 488) and Myosin IIb (Alexa 568). a) Spinning-disk-confocal image. b) Images of the same specimen using the Olympus SD-OSR spinning-disk super-resolution system. (Images courtesy of Keiju Kamijyo, Tohoku University School of Medicine, Japan.)Conclusion
Biological microscopy involves a continuous quest to image what essentially cannot be seen—the subtle and fast-changing processes of life. Super resolution provides another tool that researchers can use to learn more about the structures and dynamics of life. Each technique has its strengths and is ideally suited for a certain type of imaging, but also has factors that limit its usefulness.
The next step in super resolution may allow researchers to image fastermoving, ever-smaller structures for longer periods, even deeper within tissue. However, there will always be an object at the edge of resolution, and science will dwell on the phenomena that lie just beyond.
Russell Ulbrich is product manager, Software and Advanced Imaging Systems, Olympus America Inc., 48 Woerd Ave., Waltham, Mass. 02453, U.S.A.; tel.: 781-419-4686; e-mail: [email protected]; www.olympusamerica.com