Vast numbers of new surfactants are introduced annually for improved formulation and functionality in products ranging from personal care (e.g., soaps, shampoos, sun care, and cosmetics products), household detergents, industrial cleaning solutions, food industry items, paints, pigments, and inks to pharmaceuticals. Formulations involving surfactants as key constituents are commonly complex mixtures, and knowledge and control of surface tension are thus essential to optimize their performance. The characterization of surfactants routinely involves the measurement of the dependence of surface tension on concentration, yielding an adsorption isotherm with important properties such as surface excess concentration and critical micelle concentration revealed by these data.
The proper and cost-effective development of surfactant formulations and surface-active compounds requires not only knowledge of the properties of the various individual components, but also an understanding of mixtures and two or more surfactants and formation of mixed micelles. One characteristic of an interface is its anisotropy, which results in increased molecular organization and electrostatic fields from adsorbing ions, making it virtually impossible to accurately predict properties of mixtures. Moreover, rational development requires screening of a variety of compositions in a broad range of chemical environments, such as varying pH and ionic strength.
While surface tension measurements have traditionally been used in pharmaceutical R&D for preformulation tasks, the surface activity properties of drugs have been shown to correlate with their absorption, distribution, metabolism, excretion, and toxicity (ADME/tox),1–4 opening a novel application area for surface tension measurement in the prediction of these in vivo properties on the basis of in vitro physicochemical profiling. Scanning a large range of conditions requires enormous amounts of data. Conventional surface tension measurement is limited by slow throughput and high material consumption. This application note describes a multichannel tensiometer that is used to study the dependence of surface activity on solution composition. It makes surface tension measurement practical for modern industrial R&D.
Methods
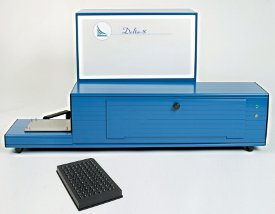
Figure 1 - Delta-8 instrument and 96-well microplate.
The Delta-8 multichannel tensiometer (Kibron Inc., Helsinki, Finland) (Figure 1) is based on the maximum pull force method, i.e., the maximum force exerted by surface tension is recorded as the wetting probe is withdrawn from the interface. This is an established, reliable, and well-documented technique.5–8 The main forces acting on a probe are the buoyancy due to the volume of liquid displaced by the probe and the mass of the meniscus adhering to the probe (Figure 2a). In the instrument, the probe is immersed approx. 1 mm into the solution and is then slowly withdrawn. The receding contact angle on the probe is effectively zero. The maximum pull force is recorded when the buoyancy force reaches its minimum, i.e., just before the meniscus breaks (Figure 2b).
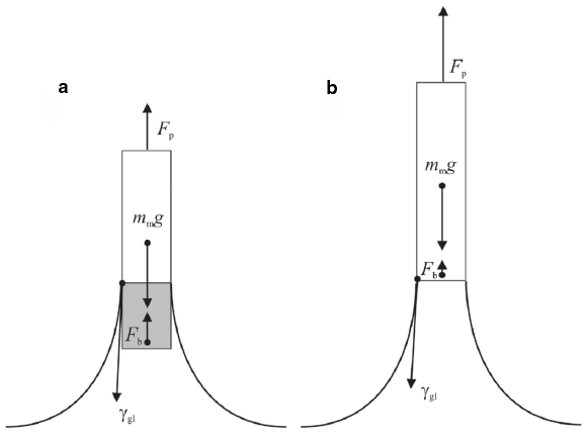
Figure 2 - Schematic illustration of measurement principle and the forces acting on the probe in a gas–liquid interface. Fp is the force acting on the probe, Fb is the lift due to buoyancy (weight of the displaced liquid), γgl is the surface tension, mm is the weight of the meniscus under the probe (the volume displaced by the probe is included in the meniscus), and γ is the contact angle between the probe and the solution being measured. The latter is negligible in most cases.
Instrumentation
At the core of the Delta-8 is a sensor consisting of eight parallel ultrasensitive microbalances positioned to match the wells of a standard 96-well plate. While the sensitivity of the balances exceeds 1.6 μg, due to practical limitations, the resolution of the instrument has been set at 0.1 mN/m (corresponding to 16 μg). Another important feature of the balance is its fast dynamic response. The measurement time is limited by the physical properties of the sample, such as viscosity, and not the properties of the balance.
Table 1 - Comparison of techniques used for the measurement of surface tension of gas–liquid interface
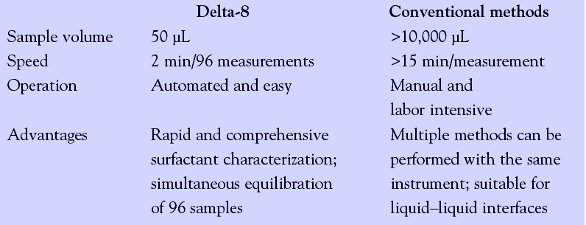
Each measurement cycle begins with cleaning the probes by heating to approx. 1000 °C for 10 sec in an electric resistor oven. This procedure was found to be effective for removing residual organic compounds that may be adsorbed on the probes after the previous measurement cycle. Importantly, uncertainties of manual probe cleaning are eliminated. The small mass of the probes allows them to cool rapidly, and hence the measurement can be initiated 10 sec after heating.
While the precision and resolution of the maximum pull force technique employed in the instrument are similar to traditional approaches using the du Nouy ring, Wilhelmy plate, or bubble pressure, there are also significant differences (Table 1). Specifically, the most serious limitation of conventional instruments is their slow speed, need for manual labor, and considerable sample consumption. Delta-8 technology eliminates these drawbacks by automation, miniaturization, and the parallel use of eight channels.
Sample preparation
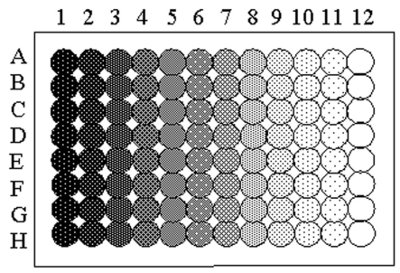
Figure 3 - Schematic of allocation of samples in the 96-well plate for the measurement of surface tension by Delta-8. (See text for details.)
Perhaps the most important application for the instrument is the measurement of surface tension versus concentration isotherms, providing an adsorption isotherm. A series of concentrations can easily be prepared by dilution across a 96-well plate, where the concentration is decreased by a dilution factor for every column using a transfer-and-mix protocol. The dilution factor describes the volumetric ratio of the transferred volume to the volume of buffer initially applied to the well and can range between 0 and 1. Using 96-well plates, the procedure yields eight parallel dilution series, each containing 12 dilutions (Figure 3). The procedure can be used in both manual and robotic sample preparation. The plate is measured after an equilibration time necessary to achieve equilibrium between the surface and bulk phases. To minimize carryover, the wells are measured in order of increasing surfactant concentration.
Results and discussion
The Delta-8 produces physicochemical data that allow the prediction of the behavior of a compound, such as a pharmaceutical, in a real biological system. For example, the data can be used to provide a rational basis for the behavior of clomipramine (Sigma-Aldrich, St. Louis, MO) after oral delivery in the gastrointestinal tract. The following example demonstrates use of the instrument to determine the behavior of an amphiphilic drug as a function of pH.
A serial dilution of clomipramine in dimethylsulfoxide (DMSO) was prepared using the transfer-and-mix procedure so that the concentrations were C0 × 500 × 0.5(n–1) mM, where n ranges from 1 to 11. C0 is a factor describing the relative concentration of the stock solutions, and was 1, 0.9, 0.8, or 0.7. Buffer solutions were made in purified water (Millipore Corp., Molsheim, France) and contained 114 mM NaCl and 50 mM Tris (J.T. Baker, Deventer, Holland). The pH was adjusted to the indicated values (7.0, 7.5, 8.0, 8.5, and 9.2) with concentrated HCl and NaOH.
In the study, because each adsorption isotherm expanded over four rows on a 96-well plate, two different buffer conditions were able to fit on one plate. Five microliters of each diluted sample was transferred into a well of rows A–D and E–H in a 96-well detection plate (Kibron), and 45 μL of buffer was added to rows A–D and E–H, with the contents mixed thoroughly. All five isotherms were prepared from the same dilution series as explained above. The plates were allowed to equilibrate for 10 min under a lid (to minimize evaporation) prior to assay.
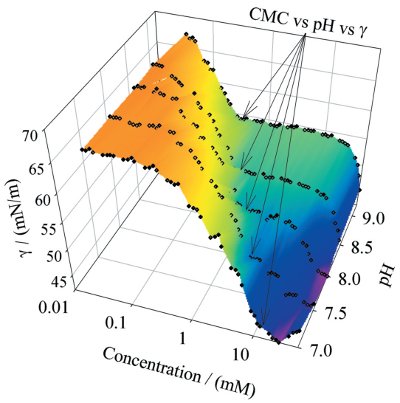
Figure 4 - Surface tension for clomipramine as a function of concentration and pH. (See text for details.)
Clomipramine is a tertiary amine with a pKa of approx. 9 and is therefore neutral at high pH and protonated at low pH. The differences in solvation of the neutral and cationic species are reflected in the critical micelle concentration (CMC)/solubility (Figure 4). The neutral form is more surface active since it is less readily solvated than the cationic form. Therefore, the onset of surface activity increases with pH. At a higher pH, micelle formation is favored due to reduced coulombic repulsion between the head groups. In the stomach, with acidic pH, the amine group becomes protonated, and hence its solubility increases while the surface activity decreases. When the compound moves into the small intestine, where the pH is considerably higher, the solubility decreases and the surface activity increases. Bile salts are excreted into the duodenum, creating an anisotropic micellar environment with a hydrophilic surface and hydrophobic core. As a result, the hydrophobic parts of clomipramine become buried in the micelle interior, while the hydrophilic amine remains on the surface, in contact with the surrounding aqueous environment. This increases the apparent solubility, which is crucial for the adsorption of many poorly soluble compounds.
Conclusion
The applications of the Delta-8 are not limited to pharmaceuticals. Assays for any surfactant-based application are easy to set up on the 96-well plates by varying different parameters along various axes of the plate, producing great amounts of data in a normal workday. The value of the instrument is not only the high throughput, but also the versatility of the multiple-well platform, which permits rapid screening of interactions in surfactant mixtures in a multitude of conditions, ultimately making extrapolations to relate the properties of surfactant to product performance. With conventional surface tension instruments, similar tasks would require weeks of intensive laboratory work. Another important factor is the low sample consumption, which makes surface activity studies affordable, even in early R&D.
References
- Suomalainen P, Johans C, Söderlund T, Kinnunen PKJ. Surface activity profiling of drugs applied to the prediction of blood–brain barrier permeability. J Med Chem 2004; 47(7):1783–8.
- Onishi Y, Hirano H, Nakata K, et al. High-speed screening and structure–activity relationship analysis for the substrate specificity of P-glycoprotein. Chem-Bio Informatics J 2003; 3(4):175–93.
- Seelig A, Gottschlich R, Devant RM. A method to determine the ability of drugs to diffuse through the blood–brain barrier. Proc Natl Acad Sci USA 1994; 91:68–72.
- Fischer H, Gottschlich R, Seelig A. Blood–brain barrier permeation: molecular parameters governing passive diffusion. J Mem Biol 1998; 165:201–11.
- Christian SD, Slagle AR, Tucker EE, Scamehorn JF. Inverted vertical pull surface tension method. Langmuir 1998; 14(11):3126–8.
- Harkins WD, Jordan HF. A method for the determination of surface and interfacial tension from the maximum pull on a ring. J Am Chem Soc 1930; 52(5):1751–72.
- Freud BB, Freud HZ. A theory of the ring method for the determination of surface tension. J Am Chem Soc 1930; 52(5):1772–82.
- Padday JF, Pitt AR, Pashley RM. Menisci at a free liquid surface: surface tension from the maximum pull on a rod. J Chem Soc, Far Trans I 1974; 71(10):1919–31.
Dr. Johans, Mr. Palonen, and Dr. Suomalainen are with Kibron Inc., P.O. Box 141, FIN-00171 Helsinki, Finland; tel.: +358 9 6811 9226; fax: +358 9 6811 9222; e-mail: [email protected]. Dr. Kinnunen is with Helsinki Biophysics and Biomembrane Group, Institute of Biomedicine, University of Helsinki, Helsinki, Finland.