Ever since chemists began using separating funnels to isolate compounds by partitioning, they have understood the potential benefits of liquid/liquid chromatography, known today as countercurrent chromatography (CCC). Yet despite this knowledge, solid/liquid chromatography techniques, such as HPLC or flash, have become the workhorses of purification. Until recently, CCC was primarily a technique for natural products or academic research and was hardly used in mainstream purification. Unfortunately, early CCC instrumentation was poorly engineered and suffered from slow speed of separation, a combination that led to negligible adoption as a complementary and orthogonal chromatography technique.
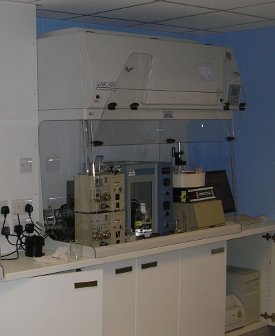
Figure 1 - HPCCC instrument.
However, a new generation of high-performance countercurrent chromatography (HPCCC) instrumentation (see Figure 1) has led to the rebirth of liquid/liquid chromatography in the 21st century. This paper discusses the development and application of these instruments, and the benefits that CCC offers to the chemist.
CCC can significantly improve a chemist’s productivity and separate compounds that were previously very difficult to isolate or uneconomical to produce. Due to the large difference in accessible stationary phase between liquid/liquid to solid/liquid chromatography—typically 70–80% compared to 5–10%—the loadings are dramatically higher, shortening the number of sample injections needed to process a batch. Furthermore, because both mobile and stationary phases are liquids, we gain two further important productivity benefits.
First, sample solubility issues are reduced because one’s options for injecting sample onto the column have been tripled. Using CCC, one can inject sample into either of the individual mobile or stationary phases or a mixture of the two, whichever combination provides the highest loading per injection. The use of two liquids is also beneficial once the sample is on the column, because even if the sample crashes out of solution, it does not cause the column to block, stopping the chromatography.
Another productivity benefit is that with CCC there is no possibility of irreversible adsorption occurring either onto or into the stationary phase. Recoveries are always very high, and it is certain that the entire sample will elute from the column.
With all of the advantages that CCC can bring to the productivity of chemists, why has it been so poorly adopted?
Poor performance and implementation
Introduced in the early 1980s, the first generation of CCC instruments were known as high-speed countercurrent chromatography (HSCCC) machines1 and were poorly adopted for three reasons. The first was speed of separation—HSCCC instruments perform separations over a period of hours, rather than the tens of minutes typical for HPLC. Secondly, the instrumentation was unreliable and therefore scientists quickly became hesitant to risk their valuable compounds. Finally, the range of equipment available was poor and typically only available at the preparative scale, requiring gram-size sample injections. This is a problem for chemists working in small-molecule synthetic chemistry, who initially may have had samples available only in hundreds of milligram amounts that took months to produce. Therefore, the entire quantity of a valuable sample would have to be injected—a risk a chemist is reluctant to take.
The combination of these factors ensured that CCC was only used as a technique of last choice, rather than adopted as a complementary and orthogonal liquid chromatography technique that could dramatically impact the productivity of chemists.
Understanding the operation of CCC instruments
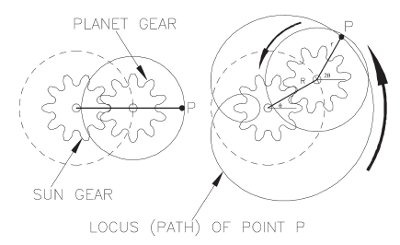
Figure 2 - Planetary motion of a J-type CCC instrument.2
All CCC instruments purify samples by the principle of partitioning, where both mobile and stationary phases are liquid and immiscible. The column is a coil of tubing wound onto a bobbin that is attached to the planet gear of the CCC instrument, which rotates around a stationary sun gear; hence, the column rotates in planetary motion. The motion of the bobbin is shown in Figure 2. This motion causes the solvents contained within the column to be subjected to varying velocities and centripetal accelerations, and allows the stationary phase to be kept within the column while permitting the mobile phase containing the sample components to be pumped past the stationary phase.
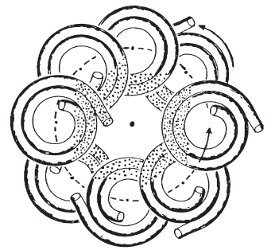
Figure 3 - Mixing and settling zones in the CCC column.1
The velocities and accelerations also cause alternating zones of mixing and settling within the column. Mixing is observed in portions of the coil closest to the solar axis, represented by the black dots. Settling of the solvents into distinct layers is observed in regions of the columns furthest away from the solar axis. This is represented in Figure 3.
A sample is introduced to the column containing the stationary phase, and as the mobile phase passes through the column, it sees alternating mixing and separating zones. This causes the sample to partition between the mobile and stationary phases, hence separating the different components of the sample. Ito3 provides an introduction to the early development of CCC instruments.
Improving CCC instrument performance
A purification process that takes hours to perform is unacceptable; thus work commenced in the mid-1990s to improve the operating performance. First, however, the key factors that determine separation performance had to be understood. Since CCC works by partitioning, the separation of different components of a sample is dependent on their partitioning coefficients (D). Component A will elute from the column after time tA and is dependent on the mobile phase flow rate (ƒm) and the stationary phase retention (Sƒ). The time of elution is represented by Eq. (1):

In the equation above, VC is the column volume. Therefore, by increasing the mobile phase flow rate (ƒm), we reduce the elution time of component A. If ƒm increases by 10, then the elution time will reduce significantly.
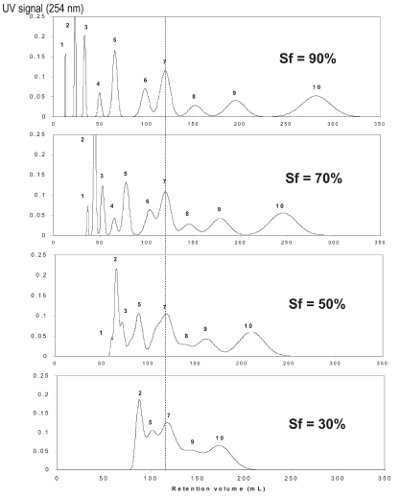
Figure 4 - Variation of resolution with stationary phase retention.
Figure 44 shows the impact on the resolution of a separation when stationary phase retention (Sƒ) is varied. This variation in stationary phase retention, at a given mobile phase flow rate, was achieved by varying the speed of rotation and hence g-level the CCC instrument generates. This demonstrates that the higher the g-level generated, the greater the resolution; however, if mobile phase flow rate is increased, then stationary phase retention will decrease. Therefore, to reduce the elution time by a factor of 10, we must increase mobile phase flow; however, by doing this, the stationary phase retention will decrease, causing a deterioration of the resolution. To compensate, we must increase the g-level generated.
Eqs. (2) and (3)2,5 show the design aspects that need to be considered to achieve the desired stationary retention and hence resolution of separation when working at higher flow rates:

Eq. (2)shows that if the mobile flow rate (ƒm) is increased by a factor of 10, the g-level (Rω2) will also need to be increased by a factor of 10 to maintain the stationary phase retention (Sƒ). In this equation, R is the rotor radius of the CCC instrument and ω is the rotational speed of the instrument.

Eq. (3) shows that if the mobile phase flow rate was increased by a factor of 10, the bore (dC) of the column would need to be increased by a factor of 1.78 to maintain the same stationary phase retention. Therefore, to dramatically improve the speed of separation, CCC instruments need to be designed to generate higher g-levels, by increasing rotational speed, combined with increasing the diameter of the column.
Development of high-performance countercurrent chromatography (HPCCC)
To rotate CCC instruments at higher speeds, the main engineering problem to overcome was the heat generated in the instrument’s bearing—hence the cooling fins, item 36, shown in Figure 5 taken from DE patents EP03715124.8, US10/958173, and 200405700-6.
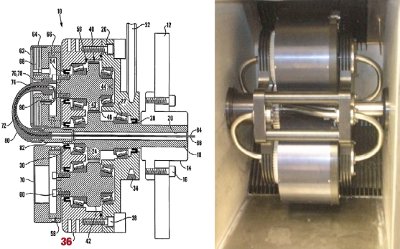
Figure 5 - Cooling fins.
The other reliability issues resolved by HPCCC instruments include: no need to bolt machines to the bench, rewind columns after a few runs, repair flying leads during a separation, or work in the back room because colleagues cannot tolerate the unit’s noise.
Below is a short summary of a seven-year R&D process that produced HPCCC instruments that generated 240 g, compared to the 80 g of the HSCCC machines. This increase in g-level and larger bore of the column has enabled a tenfold increase in mobile phase flow rate while maintaining the required stationary phase retention to ensure resolution. To demonstrate this tenfold increase in performance, Table 1 shows a comparison of the separation of honokiol and magnolol, two isomers from traditional Chinese medicines.6
Table 1 - Separation of two isomers isolated from traditional Chinese medicines
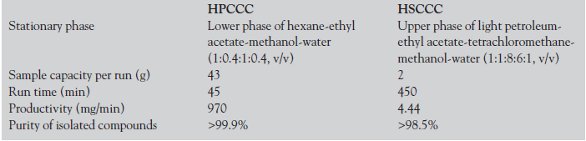
Other benefits of higher g-level CCC instruments
Having broken through the 240-g barrier, two further discoveries were made. The first is that it was now possible to develop robust analytical-scale instruments, using small-bore columns, so that milligram quantities of compound could be processed. Previously, with 80-g instruments, analytical scale had been impossible, because at that g-level the wall effects of small-bore columns affected the partitioning mechanism.7 Overcoming this hurdle allowed the technique to be used in medicinal chemistry.
The second was that with a small-scale instrument, work could begin to understand the scaleup between instruments. It was discovered that scaleup was simply volumetric and linear between all scales developed (5- to 18,000-mL columns).8
The two examples shown in Figure 6 demonstrate this volumetric scaleup. Each of these separations was first performed on the analytical-scale column at a sample volume of 300 mg, and then simply scaled up by multiplying the sample volume and the mobile phase flow rate by 26, the volume ratios between the analytical and preparative columns.
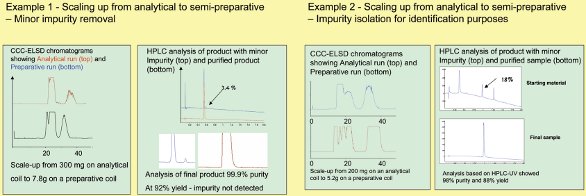
Figure 6 - Two examples showing volumetric scaleup.
Benefits of HPCCC for chemists
HPCCC can improve the productivity of chemists at all scales. It is a technique that can be applied across the whole range of polarity and to both small and large synthetic molecules, peptides, and natural products. Because HPCCC is a high-capacity technique, it is becoming the first choice for scientists when they need to produce large quantities of target compounds (i.e., high-purity product for studies in Phase 1, 2, or 3 clinical trials, or for impurities, normally found in low concentration, that need to be produced in quantity for standards).
This is especially attractive when a compound and its analogues are identified as a lead candidate required in ever-increasing quantities as they progress through the pharmaceutical development process. Using HPCCC instruments, chemists are able to concentrate on their product development process, not purification/chromatography redevelopment, as scale increases.
Performing scaleup of a purification between different sizes of HPCCC instruments is quick and simple. One simply uses the volumetric ratio between the two column volumes one wishes to use to determine the new sample volume and mobile phase flow rate.
A further significant benefit concerns sample solubility. Rather than the solubility of samples becoming a limiting factor, they tend toward irrelevance because the sample can be injected onto the column in either the mobile, stationary, or a mixture of both phases, without affecting the performance of the chromatography. This is shown in Figure 6. Solubility is far less an issue when working with liquid/liquid chromatography, and does not limit the throughput one needs to achieve.
Conclusion
Chemists and separation scientists are now able to use HPCCC in their laboratoriess and use high-capacity separation instruments on the benchtop. It is possible with pumps that work at 50 mL/min to easily process up to 200 g of crude material per day and potentially up to 400 g. This is a significant advance in reducing the chromatography bottleneck caused by the throughput constraints of liquid/solid chromatography techniques or the solubility of samples. HPCCC instruments can help solve these problems.
References
- Conway, W.D. Countercurrent Chromatography Apparatus Theory & Applications; VCH Publishers: New York, NY, 1990.
- Wood, P., Ph.D. thesis. Brunel University: Uxbridge, Middlesex, U.K., 2002.
- Ito, Y. Separation Purification Rev.2005, 34, 131–4.
- Comprehensive Analytical Chemistry; Berthod, A., Ed.; Elsevier: Amsterdam, 2002; Vol. XXXVIII, pp. 29, 200.
- Wood, P.; Janaway, L.; Hawes, D.; Sutherland, I.A. J. Liq. Chrom. Rel. Tech. 2003, 26(9,10), 1373–96.
- Keay, D. GIT Lab. J. Apr 2007, 11, 33–5.
- Janaway, L.; Hawes, D.; Ignatova, S.; Sutherland, I.A.; Wood, P. J. Liq. Chrom. Rel. Tech. 2003, 26(9,10), 1345–54.
- Wood, P.; Ignatova, S.; Janaway, L.; Keay, D.; Hawes, D.; Garrard, I.; Sutherland, I.A. J. Chromatogr. A 2007, 1151(1,2), 25–30.
Mr. Keay is Business Development Director, and Dr. Wood is Engineering Manager, Dynamic Extractions Ltd., 890 Plymouth Rd., Slough, Berkshire, U.K.; tel.: +44 1753 696979; fax:+44 1753 696976; e-mail: david.keay@dynamicextractions. com. U.S. contact: Mr. Bob Nairn; tel.: 609-332-5377; e-mail: [email protected].