High-performance liquid chromatography (HPLC) has proven to be the predominant
technology used in laboratories
worldwide during the past 30-plus
years. One of the primary drivers for the
growth of this technique has been the
evolution of packing materials used to
effect the separation. The underlying
principles of this evolution are governed
by the van Deemter equation, with
which any student of chromatography is
intimately familiar.1 The van Deemter
equation is an empirical formula that
describes the relationship between linear
velocity (flow rate) and plate height
(HETP or column efficiency). Since
particle size is one of the variables, a van
Deemter curve can be used to investigate
chromatographic performance.
As illustrated in Figure 1, as the particle
size decreases to less than 2.5 μm,
not only is there a significant gain in
efficiency, but the efficiency does not
diminish at increased flow rates or linear
velocities. By using smaller particles,
speed and peak capacity (number
of peaks resolved per unit time) can be
extended to new limits, termed Ultra Performance Liquid Chromatography
or UPLC™ (Waters Corp., Milford,
MA). Using UPLC, it is possible to
take full advantage of chromatographic
principles to run separations
using shorter columns and/or higher
flow rates for increased speed with
superior resolution and sensitivity. Figures
2 and 3 further illustrate UPLC in
action. With UPLC, compromises are
no longer necessary; in Figure 2 a separation
of eight diuretics is accomplished
in under 1.6 min. The same
separation on a 2.1 × 100 mm, 5-μm
C18 HPLC column yields almost identical
resolution, but takes 10 min. For
some analyses, however, speed is of
secondary importance; peak capacity
and resolution take center stage. Figure
3 shows a peptide map in which
the desired goal is to maximize the
number of peaks. In this application,
the increased peak capacity (number
of peaks resolved per unit time) of
UPLC dramatically improves the quality
of the data, resulting in
a more definitive map.
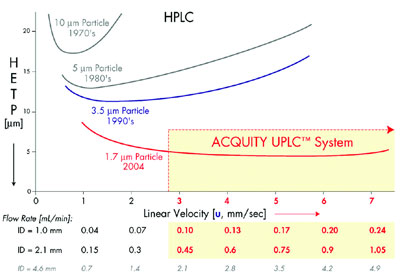
Figure 1 - van Deemter plot illustrating the evolution of particle sizes over the last three decades.
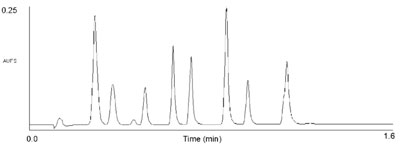
Figure 2 - UPLC separation of eight diuretics. Column:
2.1 × 30 mm, 1.7-μm ACQUITY UPLC BEH C18 at 35
°C. A 9–45%B linear gradient over 0.8 min, at a flow rate of
0.86 mL/min, was used. Mobile phase A was 0.1% formic
acid; B was acetonitrile. UV detection was at 273 nm. Peaks
are in order: acetazolamide, hydrochlorothiazide, impurity,
hydroflumethiazide, clopamide, trichlormethiazide, indapamide,
bendroflumethiazide, and spironolactone; 0.1 mg/mL
each in water.
Chemistry of small particles
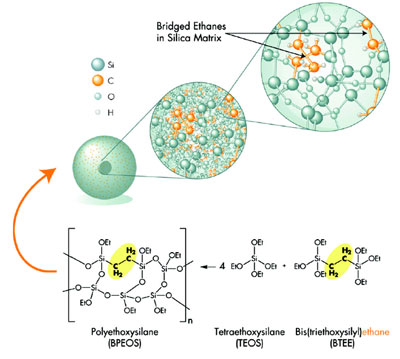
Figure 3 - Synthesis and chemistry of ACQUITY BEH 1.7-μm particles for UPLC.
The design and development
of sub-2-μm particles
is a significant challenge,
and researchers have been
active in this area for some
time to capitalize on their
advantages.2,3 Although
high-efficiency, nonporous
1.5-μm particles are commercially
available, they suffer
from poor loading capacity
and retention due to low
surface area. Silica-based particles have
good mechanical strength, but can suffer
from a number of disadvantages, which
include a limited pH range and tailing of
basic analytes. Polymeric columns can
overcome pH limitations, but they have
their own issues, including low efficiencies
and limited capacities.
XTerra® (Waters Corp.), a first-generation
hybrid chemistry that took
advantage of the best of both the silica
and polymeric column worlds, was
introduced in 2000. XTerra columns
are mechanically strong, with high
efficiency, and operate over an
extended pH range. They are produced
using a classical sol-gel synthesis
that incorporates carbon in the form of
methyl groups. In order to provide the
kind of enhanced mechanical stability
UPLC required, however, a second-generation
bridged ethane hybrid
(BEH) technology was developed: ACQUITY BEH (Waters Corp.).
ACQUITY BEH 1.7-μm particles
derive their enhanced mechanical stability
by bridging the methyl groups in
the silica matrix, as shown in Figure 4.
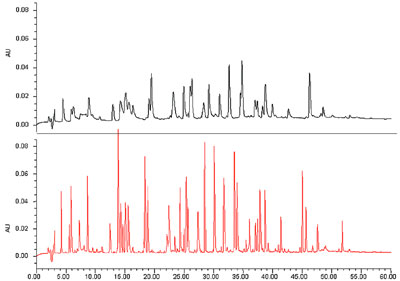
Figure 4 - HPLC vs UPLC peak capacity. In this gradient peptide map separation,
the HPLC separation (top) (on a 5-μm C18 column) yields 70 peaks, or a peak capacity
of 143, while the UPLC separation (bottom) run under identical conditions yields
168 peaks, or a peak capacity of 360, a 2.5× increase.
Packing a 1.7-μm particle in reproducible
and rugged columns was also a
challenge that needed to be overcome.
Requirements include a
smoother interior surface of the column
hardware and redesigning the
end frits to retain the small particles
and resist clogging. Packed-bed uniformity
is also critical, especially if
shorter columns are to maintain resolution
while accomplishing the goal of
faster separations. All ACQUITY
BEH columns also include eCord™
microchip technology, which captures
the manufacturing information for
each column, including the quality
control tests and Certificates of Analysis.
When used in the ACQUITY UPLC™ System (Waters Corp.), the
eCord database can also be updated
with real-time method information
such as the number of injections, or
with pressure information, to maintain
a complete column history.
Capitalizing on smaller particles
Instrument technology also had to keep
pace to truly take advantage of the
increased speed, superior
resolution, and sensitivity
afforded by smaller particles.
Standard HPLC
technology simply does
not have the horsepower
to take full advantage of
sub-2-μm particles. A new
system design with
advanced technology in
the pump, autosampler, detector, data system, and
service diagnostics was
required. The ACQUITY UPLC System has been
holistically designed for
low system and dwell volume
to take full advantage
of low-dispersion and
small-particle technology.
Achieving small-particle,
high-peak-capacity
separations requires a greater pressure
range than that achievable by today’s
HPLC instrumentation. The calculated
pressure drop at the optimum
flow rate for maximum efficiency
across a 15-cm-long column packed
with 1.7-μm particles is approx. 15,000
psi. Therefore, a pump capable of
delivering solvent smoothly and reproducibly
at these pressures that can
compensate for solvent compressibility
and operate in both the gradient and
isocratic separation modes is required.
Sample introduction is also critical.
Conventional injection valves, either
automated or manual, are not
designed and hardened to work at
extreme pressure. To protect the column
from experiencing extreme pressure
fluctuations, the injection process
must be relatively pulse free. The
swept volume of the device also needs
to be minimal to reduce potential
band spreading. A fast injection cycle
time is needed to fully capitalize on
the speed afforded by UPLC, which in
turn requires a high sample capacity.
Low-volume injections with minimal
carryover are also required to realize
the increased sensitivity benefits.
With 1.7-μm particles, half-height
peak widths of less than 1 sec are
obtained, posing significant challenges
for the detector. In order to accurately and reproducibly integrate an analyte
peak, the detector sampling rate must
be high enough to capture enough
data points across the peak. In addition,
the detector cell must have minimal
dispersion (volume) to preserve
separation efficiency. Conceptually,
the sensitivity increase for UPLC
detection should be 2–3 times higher
than HPLC separations, depending on
the detection technique. MS detection
is significantly enhanced by
UPLC; increased peak concentrations
with reduced chromatographic dispersion
at lower flow rates (no flow splitting)
promote increased source ionization
efficiencies.
Shown in Figure 5, the ACQUITY
UPLC System consists of a binary solvent
manager, sample manager (including
the column heater), detector, and
optional sample organizer. The binary
solvent manager uses two individual
serial flow pumps to deliver a parallel
binary gradient. There are built-in solvent
select valves that let the user
choose from up to four solvents. There
is a 15,000-psi pressure limit (approx.
1000 bar) to take advantage of the sub-2-μm particle in the linear velocity per
the van Deemter curve. The sample
manager also incorporates several technology
advancements. Low dispersion
is maintained through the injection
process using pressure assist sample
introduction, and a series of pressure
transducers facilitate self monitoring
and diagnostics. Needle-in-needle sampling
improves ruggedness, and a needle
calibration sensor increases accuracy.
Injection cycle time is 25 sec
without a wash and 60 sec with a dual
wash used to further decrease carryover.
A variety of multiple-well plate formats
(deep-well, mid-height, or vials) can
also be accommodated in a thermostatically
controlled environment. Using
the optional sample organizer, the sample
manager can inject from up to 22
multiple-well plates. The sample manager
also controls the column heater.
Column temperatures up to 65 °C can
be attained. A “pivot out” design provides
versatility to allow the column outlet to be placed in closer
proximity to the source
inlet of an MS detector to
minimize sample dispersion.
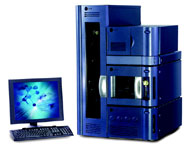
Figure 5 - ACQUITY UPLC System.
The ACQUITY UPLC Tunable UV (TUV) Detector
(Waters Corp.) includes
new electronics and
firmware to support Ethernet
communications at the
high data rates necessary for
UPLC detection. Conventional
absorbance-based
optical detectors are concentration
sensitive; for
UPLC use, the flow cell volume
would have to be
reduced in standard UV-VIS
detectors to maintain
concentration and signal.
Smaller-volume conventional
flow cells would also
reduce the pathlength upon
which the signal strength depends
(recall Beer’s Law). Worse, a reduction
in cross-section means the light path is
reduced and transmission drops, increasing
noise. Therefore, if a conventional
HPLC flow cell is used, UPLC sensitivity
would be compromised. The
ACQUITY UPLC TUV detector cell
consists of a light-guided flow cell
equivalent to an optical fiber. Light is
efficiently transferred down the flow cell
in an internal reflectance mode that still
maintains a 10-mm flow cell pathlength
with a volume of only 500 nL. Tubing
and connections in the system are efficiently
routed to maintain low dispersion
and to take advantage of leak
detectors that interact with the software
to alert the user to potential problems.
Applications
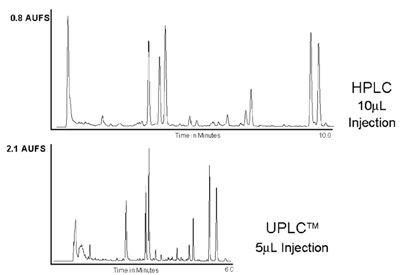
Figure 6 - Comparison of HPLC and UPLC for the separation
of a ginger root extract. HPLC conditions—Column:
2.1 × 100 mm, 5.0-μm prototype BEH C18 at 28 °C. A
25–96%B linear gradient over 10 min, at a flow rate of 1.0
mL/min, was used. Mobile phase A was water; B was acetonitrile.
UV detection was at 230 nm, 10-μL injection. UPLC
conditions—column: 2.1 × 100 mm, 1.7-μm ACQUITY
BEH C18 at 28 °C. A 50–100%B linear gradient from 1.4
to 3.7 min followed by a hold until 6.0 min, at a flow rate of
0.3 mL/min, was used. Mobile phase A was water; B was
acetonitrile. UV detection was at 230 nm, 5-μL injection.
Chromatographers are used to making
compromises, and one of the most common scenarios involves sacrificing
resolution for speed. In addition,
for complex samples such as
natural product extracts, added resolution
can provide more information
in the form of additional peaks.
Figure 6 shows an HPLC versus
UPLC separation comparison of a
ginger root extract sample where
both speed and resolution are
improved, as well as an increase in
sensitivity. DryLab software (Rheodyne,
Rohnert Park, CA) was used to
model and redevelop the separation
and transfer it to the ACQUITY
UPLC System and BEH chemistry.
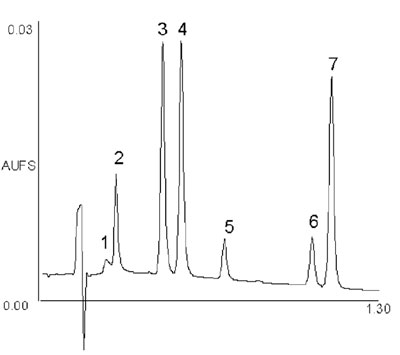
Figure 7 - UPLC separation of seven coumarins illustrating
fast method development. Column: 2.1 × 30 mm, 1.7-μm
ACQUITY UPLC BEH C18 at 35 °C. A 20–40%B linear
gradient over 1.0 min, at a flow rate of 0.86 mL/min, was
used. Mobile phase A was 0.1% formic acid; B was acetonitrile.
UV detection was at 254 nm and 40 pts/sec. Peaks are in
order: 7-hydroxycoumarin-glucuronide, 7-hydroxycoumarin,
4-hydroxycoumarin, coumarin, 7-methoxycoumarin,
7-ethoxycoumarin, and 4-ethoxycoumarin.
Faster separations can lead to higher
throughput and time savings when
running multiple samples. However, a
significant amount of time can also be
consumed in developing the method
in the first place. Faster, higher-resolution
UPLC separations can cut
method development time from days
to hours or even minutes. Figure 7 is
an example of a UPLC separation of
several closely related coumarins and
a metabolite that was developed in
under 1 hr, including UPLC scouting
runs for gradient optimization and
individual runs for elution order identification.
These runs were performed
in a fraction of the time that would be necessary with conventional
HPLC, resulting in significant
time savings in the
method development laboratory.
Conclusion
At a time when many scientists
have reached separation
barriers pushing the limits of
conventional HPLC, UPLC
presents the possibility to
extend and expand the utility
of this widely used separation
science. New ACQUITY
UPLC technology in chemistry
and instrumentation provides
more information per
unit of work as UPLC begins
to fulfill the promise of
increased speed, resolution,
and sensitivity predicted for
liquid chromatography.
References
- van Deemter JJ, Zuiderweg FJ, Klinkenberg A. Chem Eng Sci 1956; 5:271.
- Jerkovitch AD, Mellors JS, Jorgenson JW. LC·GC North America 2003; 21:7.
- Wu N, Lippert JA, Lee MA. J Chromotogr 2001; 911:1.
Dr. Swartz is Principal Consulting Scientist, and
Mr. Murphy is Manager, Corporate Communications,
Waters Corp., 34 Maple St., Milford, MA
01757, U.S.A.; tel.: 508-482-2742; fax: 508-482-3085; e-mail: [email protected].
The authors would like to acknowledge the contributions
of the ACQUITY program team at
Waters, particularly Eric Grumbach, Pat
McDonald, Michael Jones, and Marianna Kele,
for their contributions to this manuscript.