The performance of solid pharmaceutical products is normally assessed using the pharmacopeial in vitro dissolution test. The dissolution test is used both to ensure quality and consistency of investigational clinical and registered commercial materials, and as a tool to assist in the development of new products.
In recent years there has been considerable interest in exploring how in vitro dissolution testing can be used more effectively in the product development phase. An example of this is using more biorelevant dissolution media, such as simulated gastric fluid, with the aim of improving the connection between the product behavior observed in vitro and that in vivo. The use of clinically relevant dissolution tests has been emphasized as part of the Quality by Design (QbD) initiative, for example, in the 2006 workshop organized by the American Association of Pharmaceutical Scientists.1 It was noted at the workshop that different dissolution methods may be used for product development and quality control purposes, and that new measurement technology can be valuable in the development phase. For example, the use of fiber optics and in situ particle size measurement can provide information about the mechanism and kinetics of drug release. Such mechanistic information can help to shift the dissolution paradigm from the current data-driven approach toward a knowledge-driven perspective. Mechanistic performance knowledge will help with generating a deeper understanding of the critical product quality attributes and process parameters.
This article discusses UV imaging, a new technology that has been designed to improve our understanding of the kinetics and mechanism of drug substance release.2 The theories covering the dissolution of pure substances all stress the importance of the solution concentration gradient that exists between the solid/solution interface and the bulk solution.3 However, while this concentration gradient is central to theory, detailed experimental verification of its applicability to pharmaceutical substances has been difficult until now. The approach described here relies on the fact that most pharmaceutical drug substances contain a UV chromophore, and that UV absorbance is a sensitive and robust measurement approach. The use of an imaging detector, with spatially resolved absorbance and thus concentration data, can give information on the concentration gradient and how it changes with experimental conditions.
UV imaging technology
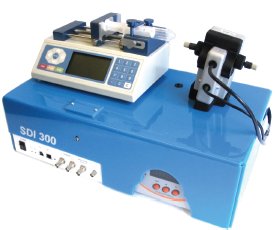
Figure 1 - Photograph of ActiPix SDI 300 dissolution imaging system.
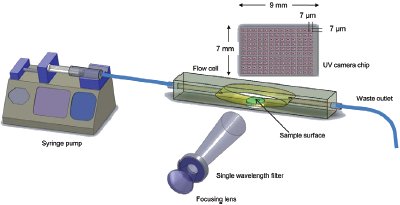
Figure 2 - Diagram of ActiPix SDI 300 dissolution system showing the sample holder, dissolution flow cell, and UV imaging detector array.
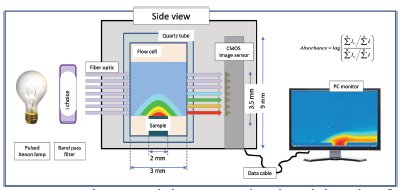
Figure 3 - Diagram of ActiPix SDI 300 dissolution imaging system showing the principle of imaging the spatially resolved local solution concentration. The solution concentration is highest closest to the surface of the dissolving solid, and this will be reflected in the greater level of UV absorbance (shown in red in the false color image).
The ActiPix SDI 300 (Paraytec Ltd., York, U.K.) comprises a sample flow cell, syringe pump, UV lamp and detector, and control and data analysis system. The system is shown in the photograph in Figure 1 and the schematics in Figures 2 and 3. The system uses a complementary metal oxide semiconductor (CMOS) image sensor (7 mm × 9 mm made up of 1.3 million 7 × 7 μm pixels) for recording light transmission through the flow cell (Figure 2). A broad-spectrum pulsed xenon lamp (PerkinElmer, Waltham, MA) provides flashes of light that are synchronized so that light does not strike the pixels during the detector readout phase (Figure 3). Because a broad-spectrum lamp is used, each pulse is focused onto a narrow bandpass filter (10 nm), and is then passed through a diffuser before being refocused onto a 2-mm round UV fiber optic. The termination end of the fiber optic splays the fibers into a 9-mm-wide single line to effectively illuminate the entire width and most of the height of the image sensor. The lamp pulse rate is determined by the data transfer rate from the sensor to the PC, based on the number of rows and level of binning selected. The binning level is chosen to favor either spatial resolution or sensitivity, using a range of 1 × 1 (maximum resolution) up to 10 × 1. Imaging rates vary from 0.5 up to about 8 Hz, with a typical dissolution experiment using around 2 Hz. The viewing area and binning are selected by the operator. Using 0.7 Mpixels, the data file sizes are typically hundreds of MBs, but by subsampling images it is also possible to maintain reasonable file sizes in extended dissolution experiments.
Once the lamp level has been adjusted to avoid saturation, the first images collected are dark values or electronic noise. An average for every pixel is collected over a 10-sec period. The average dark value is then subtracted from each background value to negate contributions from electronic noise. The subsequent 10 sec are used to collect the background intensities from the buffer contained within the flow cell.
The intensity at each pixel is converted into an absolute absorbance by first subtracting the dark values and then applying the standard log equation from the initial transmission (I0) and the sample transmission (I). The absorbance values for each pixel in the selected viewing area create a 2-D image, and the images collected for the duration of the experiment are used to create a movie. Thus, a high-resolution 2-D movie of UV absorbance is captured that provides a detailed view of the dissolution process at the solid–liquid interface. Applying proprietary software tools then allows extraction of unique and common results of use to the pharmaceutical analyst.
The flow cell has been specially designed to provide laminar flow across the surface of a compacted active pharmaceutical ingredient (API) powder. With the sealed rectangular quartz tube pressed against the imager face, a complete side view of the powder surface, including 3 mm up and downstream and 3 mm above, comprises the total viewing area (Figure 2). The laminar parabolic flow is parallel to the image sensor but also passes across the powder with a surface velocity near zero. The API powder is compacted inside a stainless steel tube (2.0 mm i.d.) using a miniature press and torque screwdriver, and is held exactly under the fiber optic in the center of the quartz tube. Buffer is pumped into one side of the flow cell through a small orifice and is then allowed to expand in the parabolic reaction zone in order to provide laminar flow. The entire quartz tube assembly sits inside a light-tight cartridge that positions the flow cell directly over the image sensor. The heart of the flow cell is illuminated by UV light from the lamp brought in by the fiber optic. Volumetric flow originates from an accurate, stable syringe pump and typically uses a 20-mL syringe. Multiple flow rate steps are controlled using the software to synchronize the pump and the UV images. Thus, data required for determining results such as the intrinsic dissolution rate are readily available; this is discussed in more detail below.
Applications
Use of the system is illustrated by examining the dissolution behavior of a highly soluble base, the β-blocker atenolol, and a poorly soluble acid, the diuretic furosemide.
Atenolol
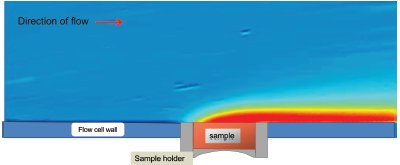
Figure 4 - False color image showing the dissolution of atenolol. The solid atenolol sample is in the center of the image, and the buffer flow is from left to right. The region of highest UV absorbance (hence highest solution concentration) is shown in red and the region of lowest absorbance is in blue.
Atenolol was packed with a torque setting of 20 cNm; Figure 4 shows the UV image produced using a pH 4.5 phosphate buffer at a flow rate of 0.6 mL/min and a 254-nm filter. The results are presented as a false color image with the areas of high UV absorbance shown in red and those of low UV absorbance in pale blue. Figure 4 shows the tube containing the compact of solid atenolol in the bottom center of the image. Above the tube and to the right, the bright red region is the plume of concentrated atenolol solution being transported away by the flowing buffer. Above the bright red and yellow regions is a pale blue area that is a result of dilute atenolol solution. The dilute atenolol solution diffuses further into the bulk buffer solution as the downstream distance increases. The left-hand side of the image is upstream of the solid atenolol and shows the inflow of fresh phosphate buffer from the syringe pump (i.e., no UV absorbance).
Furosemide
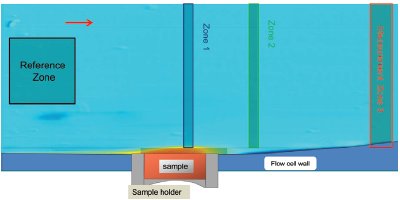
Figure 5 - False color image showing the dissolution of furosemide. The image shows the position of the solid furosemide sample, the UV absorbance reference zone, and the three solution absorbance measurement zones. Furosemide is much less soluble than atenolol, and this is reflected in the fact that significant solution concentrations are only detectable close to the surface of the solid (compare with Figure 4).
Furosemide was packed with a torque setting of 80 cNm; Figure 5 shows the UV image produced at 280 nm using a pH 2.0 phosphate buffer at a flow rate of 2.0 mL/ min. At pH 2.0, furosemide is uncharged and thus has a very low solubility in aqueous buffers. The results are again shown as a false color image with the highest absorbance depicted in red and orange. The region of high furosemide concentration is limited to the area very close to the sample surface and immediately downstream of the sample cup. This is in sharp contrast to the results for atenolol, where the much larger region of high UV absorbance reflects the much higher solubility of atenolol. The solution absorbance profile in the z-dimension (i.e., perpendicular to the flow) can be extracted from different locations in the 2-D image using the three measurement bars shown in Figure 5.
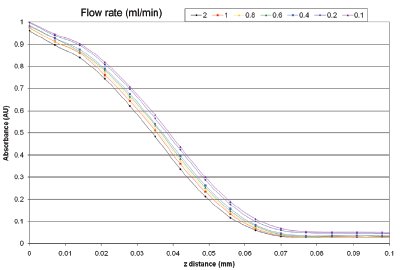
Figure 6 - UV absorbance gradient of furosemide as a function of varying buffer flow rate in the range 0.1–2.0 mL/min. The absorbance profile is taken from measurement zone 1 in Figure 5, and shows that the solution concentration is low except for close to the sample surface.
The profiles produced from the left-hand bar (i.e., directly above the dissolving solid) at a range of flow rates are shown in Figure 6. It can be seen that the absorbance (hence concentration) decreases rapidly as we move into the bulk solution perpendicular to the direction of buffer flow. The profile obtained is in accordance with the general expectations from convective-diffusion theory.3 As the flow rate is dropped, the impact of diffusion becomes more significant and furosemide is able to spread out further into the bulk solution.
Intrinsic dissolution rate
Standard intrinsic dissolution rate values of (mass/time/area) are easily calculated from the volumetric flow rate and the absorbance intensities in measurement zone 3 (see Figure 5). Once the parameters for molecular weight and extinction coefficient are entered, mass is calculated by applying Beer’s law to obtain mass per volume. The known volumetric flow rate is multiplied by the concentration to give mass/min. This result is then divided by the known sample surface area to give mass/min/area (mg/min/cm2). Furthermore, since the flow regime in this apparatus is laminar, the velocity through the measurement zone is faster at the center of the flow stream than at the surface wall (parabolic flow). To adjust for this difference, each row of pixels in the measurement zone is corrected for transit rate through the zone based on the known volumetric flow rate to provide an accurate mass transport through the measurement zone at all heights away from the surface.
Conclusion
UV imaging can provide detailed insight into the convective-transport processes that occur during the dissolution of pure drug substances. In particular, UV imaging offers information about the concentration gradient produced near the surface of the dissolving substance. The technique shows how this concentration gradient changes with spatial location, and how it is altered by the flow rate of the dissolution buffer.
References
- Tong, C.; D’Souza, S.S.; Parker, J.E.; Mirza, T. Pharm Res.2007, 24, 1603–7.
- Wren, S.; Goodall, D.; Lenke, J.; Moon, K.; Swarbrick, M.; Chapman, A. J.Pharm. Pharmacol. 2009, 61, S1, A110.
- Levich, V.G. Physicochemical Hydrodynamics; Prentice-Hall: Englewood Cliffs, NJ, 1962.
Dr.Wren is a Principal Scientist, AstraZeneca, Silk Road Business Park, Macclesfield SK10 2NA, U.K.; tel.: +44 1625 513 498; e-mail: [email protected]. Mr. Lenke is a Senior Applications Engineer, Paraytec Ltd., York, U.K.