Historically, the life sciences have been qualitative in nature, seeking to identify but seldom understand quantitatively. The development of separation technologies such as electrophoresis, chromatography, and centrifugation began as far back as the early part of the twentieth century; however, these technologies progressed very slowly and contributed only minimally, until the middle part of the last century, when instrumentation started to increase their speed and accuracy. This article will identify several important technologies now used in the life sciences that are, in fact, critical to fulfilling the potential of the biological sciences, based on the use of increasingly sophisticated instrumentation.
Electrophoresis
Electrophoresis is the movement (migration) of biomolecules under the influence of an electric field. The technique was first implemented with various anticonvention matrices, but acrylamide gels, introduced in the late 1950s, have come to dominate the field. Gel electrophoresis (sodium dodecyl sulfate-polyacrylamide gel electrophoresis [SDS-PAGE], isoelectric focusing gel electrophoresis, etc.) has become a mainstay for the qualitative analysis of biological molecules. Instrumentation for gel electrophoresis has changed only slightly over the years, and the time required to complete a gel analysis is still very long and reproducibility is poor. Gel electrophoresis techniques remain very important in the analysis of biomolecules because of their historical usage; however, only limited advances have been made or are anticipated.
Instrumentation in the form of capillary electrophoresis (CE) came to the rescue in the 1980s. Early developments, many from the laboratory of Prof. Jim Jorgenson at the University of North Carolina (Chapel Hill), helped pave the way for the introduction of commercial instrumentation in the late 1980s.1 A number of practical problems, however, such as peak distortion due to Joule heating, poor reproducibility, and detection difficulties, held back the implementation of capillary electrophoresis. Although improvements to instrumentation in the early 1990s and a better understanding of optimal separation conditions reduced Joule heating concerns, reproducibility remained a problem. Significant improvements in reproducibility have largely been a result of careful attention to analysis conditions. Because electrophoresis consists of electrolytic cells, buffers are modified under prolonged usage and buffer vials must be changed regularly. Special care must be given to injection conditions since these can affect reproducibility. The surface of the fused-silica capillary used is modified during an analysis. Careful conditioning of the capillary is essential. Lastly, internal standards are very important in maximizing reproducibility.
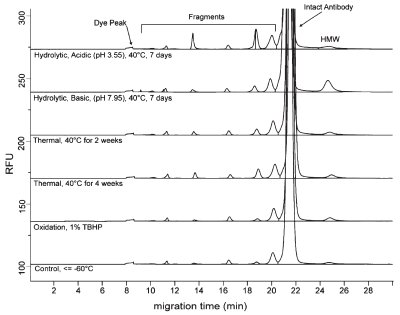
Figure 1 - Monoclonal antibodies constitute the majority of protein therapeutic drugs in the pipeline today. The instrumental form of SDS-PAGE, CE-SDS, is an important technique used to monitor monoclonal antibody stability. (Reproduced with permission from Ref. 2.)
Even with these steps, reproducibility in CE is somewhat worse than in chromatography but is much better than in gel electrophoresis. Capillary electrophoresis analogues to SDS-PAGE and isoelectric focusing gel electrophoresis are fast becoming the most important use of this instrumental technique. An example is the monitoring of monoclonal antibodies during stability testing, for which the standard approach is CE-SDS (Figure 1). The technology has advanced from capillary tubes to even smaller channels in chips. The microfluidic chips offer the promise of very rapid separations and useful quantitative assays, but materials science issues seem to restrict the applications to the few that are intensely engineered.
Chromatography
From its inception, chromatography has been interwoven with the life sciences. In fact, chromatography was initially developed in the early 1900s by Mikhail Tsvet for use in the life sciences. Tsvet was investigating plant pigments when he came upon the idea of separating plant extracts by partial filling of a glass tube with an adsorbent and passing an organic solvent through the tube after placing the plant extract solution on top of the adsorbent. Plant pigments were separated and collected for further study using this technique. Chromatography, as Tsvet labeled it, was later used in the study of dairy products and other biological samples. Amino acid analyzers based on ion exchange chromatography were developed in the 1950s as were dextran gels (Sephadex®, GE Healthcare Bio-Sciences Corp., Piscataway, NJ) used for size separations and ion exchange separations of proteins and nucleic acids. While these were effective, they were very slow and simple in their use of instrumentation and mostly qualitative in nature.
The development of high-performance liquid chromatography—the instrumental form of the chromatogrphy developed by Mikhail Tsvet in the 1960s—soon focused on life science applications and began to make the analysis of molecules of interest to life scientists quantitative. An early application of HPLC was the analysis of nucleotides and nucleosides from nucleic acids by anion exchange chromatography. These could be quantified for the first time. However, since it offered superior resolution and speed, reversed-phase HPLC (RP-HPLC) soon began to dominate this new technique and was used for many small molecules of biological interest such as pharmaceutical drugs, but had limited utility for larger biomolecules. Instrument development in the form of HPLC columns particularly suited to analysis of proteins, peptides, and other larger biomolecules extended the practical utility of instrumental HPLC to these molecules important to the biotechnology field.
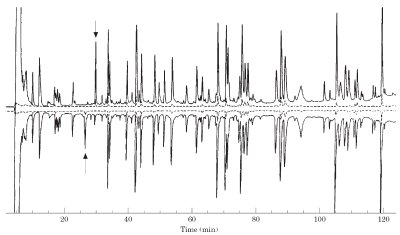
Figure 2 - Peptide maps consist of the separation of peptides from a trypsin digested protein using RP-HPLC. Peptide maps can determine genetic changes, chemical degradations such as deamidation or oxidation, or identify the sites of glycosylation (addition of polysaccharide). In this example, the top chromatogram is the peptide map of the digested protein after removal of the glycan and the bottom chromatogram is the peptide map of the native protein. The arrows point to the peptide to which the glycan is attached. Removal of the glycan causes the peptide to elute later in RP-HPLC. (Reproduced with permission from Ref. 3.)
In the 1980s, the first protein therapeutics were regulated as biological products with little quantitative data. Kathy Zoon, former director of the Center for Biologics Evaluation and Research (CBER, Rockville, MD), once said “Historically, biological products have been complex mixtures of molecular species that were difficult to characterize as individual entities. . .. Because of the limited ability to characterize the identity and structure and measure the activity of the clinically active component(s), a biological product was often defined by its manufacturing process.” Advances in analytical instrumentation, primarily in HPLC, allowed greater understanding of protein therapeutics and led to significant changes in the regulation of biotechnology products. Peptide mapping using reversed-phase HPLC (Figure 2) was applied early in the analysis and characterization of protein therapeutic drugs and proved to be of such significance that, combined with other analytical techniques, the FDA decided to implement a change in the regulatory protocols process in 1996, known as the well-characterized biologics. This resulted in regulation based upon structure, as opposed to process, culminating in the harmonization of the regulation of protein therapeutics with that of small molecules. The regulatory responsibility for protein drugs was transferred to CDER in 2002, completing the transition.