A bioscaffold is a medical device designed to support the growth of new tissue. Scaffolds are used in vivo to support the regrowth of soft tissues and bone after surgery or injury; in vitro they assist in the growth of new organs from adult stem cells. It has been found that the structure of the bioscaffold has a significant effect on the growth of the new tissue.1 Moreover, different scaffold structures are required for different tissue growth. For example, the scaffold required for a lung differs from that required for cartilage, which is different from that required for skin.
Scaffold porosity and tissue growth
The porosity of the scaffold is particularly important in achieving the optimum rate of new tissue growth. The porous nature of the sheets encourages new cell growth; as new tissue grows in the pores, the scaffold degrades, leaving only the healthy regrown tissue in its place. Achieving the optimum texture and porosity of the sheets is essential to support and encourage the body to generate new cells as quickly as possible, and to ensure the desired type of cell structure.
The production of the scaffolds therefore requires precise control over the structure of the resultant sponge to achieve a pore size that is both specific and consistent throughout the material.
Collagen scaffold production
Collagen is a protein used by the body for tissue generation, and is a major component of our connective tissue. Collagen is used widely in tissue engineering, often as a bioscaffold. It is readily available from animals, and its acellular structure can be manipulated to form porous sheets of varying structure.
Collagen scaffolds are produced as follows.2 Bovine or porcine collagen is chopped up to the required length and dissolved in a solvent such as acetic or hydrochloric acid. Water is added to create an emulsion, which is subsequently frozen. The emulsion can be frozen by placing it in a metal mold and immersing it in liquid nitrogen, but far more controlled freezing is achieved through cooling on a freeze dryer shelf. During freezing the solution forms ice crystals in the structure.
Finally, the frozen emulsion is lyophilized (freeze-dried) to remove the ice crystals, leaving a collagen sponge with the required matrix of pores. The removal of water during this stage of lyophilization results in intermolecular cross-linking between the collagen strands to form a stable collagen scaffold.
Lyophilization is commonly used to stabilize products that would otherwise quickly degrade, and is widespread in higher-value industries such as pharmaceuticals and biotechnology. The ability to avoid high temperatures makes it ideal for the preservation of sensitive tissues and biological products.
Freeze-drying holds a wealth of promise for regenerative medicine. The prospect of preserving valuable biological materials such as blood, tissues, and ultimately entire organs is leading research into how to safely freeze these delicate materials. The major issue confronting scientists is that the ice crystals formed during freezing can damage or destroy more sensitive types of cells.
In the case of bioscaffolds the holes from the ice crystals are just what are needed to create the porous structure, but there is still a requirement for a very high degree of precision in the freezing process. The successful and accurate production of bioscaffolds demands control over the pore size in the range of micrometers, and consistency throughout each sample.
Freeze-drying takes place in three stages: freezing, primary drying, and secondary drying (see Figure 1).
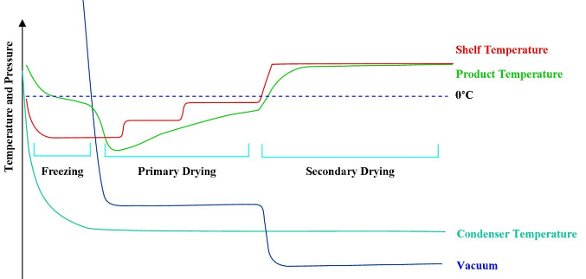
Figure 1 - Freeze-drying cycle. As a result of sublimation cooling, the product temperature is below the shelf temperature for the beginning of primary drying.
- Freezing. The first stage in lyophilization is for the sample to be frozen. The manner of freezing determines the crystalline structure, which in the case of collagen bioscaffolds is critical for its resultant structure. The challenge is to be able to control the freezing conditions sufficiently to achieve the desired result.
The pores in the final scaffold are a by-product of the freezing process—the ice crystals formed during freezing leave holes in the structure when the sample is dried. As a basic principle, fast freezing produces small crystals, while slow freezing produces larger crystals. In the case of collagen bioscaffolds, the range of desired pore size is in the micrometer range.3 For example, endothelial cells in blood vessels have been shown to grow optimally with a scaffold with a pore size of 20–80 μm, whereas osteoblasts require pores larger than 100 μm for bone formation.4–7 Therefore, control of the rate of crystal formation is critical to the successful and accurate production of bioscaffolds.
While the rate of freezing determines the pore size, the temperature gradient across the sample determines the homogeneity. Any variation in the temperature as the sample is frozen results in a change in freezing rate and therefore a variation of pore size throughout the lattice.
Annealing is a technique of raising and lowering the temperature of the crystallizing solution to change the structure of the crystals that are forming. Annealing can be used to increase the size of the ice crystals formed by up to 40%,3 providing an additional means of control over the crystal structure.
The manipulation of the freezing stage allows the pore size and surface texture of the final dried material to be tightly controlled with a high level of repeatability.
- Primary drying. Once frozen, the collagen is dried to remove the solvent by sublimation. The sample is kept below its critical temperature so that it stays in a frozen state while a moderate vacuum is applied. The pressure/temperature balance is adjusted until it reaches the triple point so that the ice sublimes straight to a vapor without going through the liquid phase.
Sublimation leads to evaporative cooling, which further lowers the sample temperature. To maintain the sample at a constant temperature, heat must be applied to compensate for sublimation cooling. Failure to do so would result in the sample cooling and the process stopping, but adding excess heat risks melt or collapse, leading to a loss of the engineered structure. The energy transfer during sublimation is therefore one of balance, where the mass of the collagen remains frozen in the required structure, while just enough heat is used to allow the change of state.
- Secondary drying. Secondary drying is a desorption process that removes any water that is chemically bound to the lattice. Desorption drying is achieved by raising the temperature of the sample and reducing the chamber pressure to a minimum. The moisture level at this stage may be around 5–10%.
Depending on how dry the end product needs to be, secondary drying may be a long and slow process. In the case of a protein such as collagen, care must be exercised. While polymerization during water removal is required to form the cross-linked structure to create a stable sponge, higher temperatures in secondary drying may result in biodegration of the sample.