Sample introduction has been a problem
for analytical chemists for decades.
In 1984, Richard Browner and Andrew
Boorn went so far as to call sample introduction
“The Achilles’ Heel of Atomic Spectroscopy.”1 Since that time, a multitude
of variations have been developed
and commercialized to address
some of the deficiencies. The most common
form in which samples are introduced
for analytical spectrometry is as
an aerosol via pneumatic nebulization.
The inherent inefficiency of the nebulizer–spray chamber combination has
been investigated by numerous authors,
and Barry Sharp presented a thorough
examination of the problem in two
papers in 1988—one on nebulizers and
a second on spray chambers.2,3 Attempts
to increase the mass-transfer
efficiency
of the sample introduction system
resulted in a wide range of solutions
such as ultrasonic nebulizers (USNs)
and high-efficiency nebulizers.
At typical flow rates, sample introduction
systems based on a nebulizer–spray chamber combination deliver
poor transport efficiencies, typically
between 1 and 3%. The optimum
strategy for improving performance
is to increase analyte transport efficiency.
Ultrasonic nebulizers are said
to improve delivery by an order of
magnitude, and nebulizers that introduce
the aerosol directly into the
plasma approach 100% efficiency,
albeit at greatly reduced flow rates.
Although solid sample introduction
techniques have been reported that
involve laser ablation, direct insertion
devices, powders, or slurries,
these methods appear to have limited
application, since most samples are
prepared in solution.
Inductively coupled plasma-atomic emission spectroscopy (ICP-AES) and ICP-MS are robust and established
techniques for elemental analysis.
These instruments are capable of providing
the required sensitivities, detection
limits, and linear dynamic ranges
for the quantitative determination
of the majority of elements required.
There are exceptions. The elements
of group 14–16 typically exhibit low
sensitivities, due in part to their relatively
high ionization potentials. An
area that continues to receive much
attention is vapor generation. Several
elements of particular concern to both
the environmental and metallurgical
communities are the so-called hydride-forming
elements (As, Sb, Bi, Ge, Sn,
Pb, Se, Te, and Hg). The transformation
of elements into volatile species
has continued to elicit interest from
both the academic community and the
contract laboratory environment.
There are several advantages to introducing
the sample as a volatile species,
and this has prompted Nakahara
to describe it as the ideal method for
sample introduction.4 The principal
advantage is the high transport efficiency
that can be achieved. Le et al.
showed that around 95% of arsenic is
removed from solution when solutions
of arsenic(III) react with borohydride.5
A second advantage is the possibility of
separating the analyte from the matrix.
In order to optimize these advantages,
especially the latter, proper design of the
phase separator is essential. One
major drawback is that the vessel
in which the hydride generation
took place has meant that applications
of the technique were
typically limited to either single-elements
determination, where
the atomic absorption technique
provides excellent results when
combined with a quartz tube
atomizer, or multielement techniques
using the ICP.6,7 Contract
laboratories are normally required
to determine and report on a
large number of elements for the
majority of samples that are analyzed.
This requirement dictates the use of a
multielement technique based on either
atomic emission or mass spectrometry in
order to provide timely results.
The use of vapor generation as a
means of sample introduction for
ICP-AES dates back to the late 1970s.
These early systems used atomic
absorption (AA)-type batch systems
for the generation of the volatile species.
Thompson et al. developed a
continuous system and proposed the
possibility of simultaneous determination
of the hydride-forming elements.
It was also realized that the multielement
capability of the ICP-AES
could further be exploited through the
simultaneous determination of conventional
and hydride-forming elements.
8 Efforts over the last two and a
half decades have provided a number
of designs that seek to combine the
nebulizing function with the vapor-generation
function into one device
in order to determine, simultaneously,
both hydride formers and nonhydride
formers. The first strategy used a dual
tandem nebulizer design.9 A reductant
solution aerosol, generated by the
first nebulizer, was introduced into the
gas orifice of the second nebulizer, to
which the sample solution was fed.
The mixed aerosol was subsequently introduced to the plasma. This
approach, as well as other systems
whereby the hydrides are formed using
a nebulizer or “detuned” nebulizer, are
nonideal, since a significant amount
of reagent is introduced to the plasma.
A second strategy involved collecting
the waste solution from the nebulized
aerosol in a reservoir formed using an
elevated drain inside the spray chamber.10 Here, the reductant solution
was pumped into the reservoir to generate
the hydride species. Hydrogen
gas, which is also formed during the
reaction, served to strip the volatile
species from solution and into the
spray chamber, where they become
entrained in the sample aerosol that
is transferred to the plasma. This basic
approach has been used several times.
Reservoir-based systems have two
primary disadvantages: sample cross-talk
cannot be avoided, and high acid
concentration is required to generate
sufficient hydrogen for adequate
stripping of the analyte. One system
has a dedicated pump channel for the
constant introduction of concentrated
hydrochloric acid to ensure adequate
hydrogen production. This extra
channel allows the sample to be prepared
at lower acid concentration, but
issues remain in regard to the cost of
the additional acid and to the safety
of handling, the consequences of a
highly corrosive environment, and the
disposal of highly acidic wastes.
A third approach was to use a manifold
system to mix the sample and reductant
together in a T, after which the mixture
is introduced into the spray chamber.11 The drawbacks associated with
this approach are described above.
A recurrent problem, and one that
has challenged many researchers over
the last three decades, has been the
rapid removal of the volatile hydrides
(and other species) from solution.
Early designs of the gas–liquid separator
relied on high acid concentrations
to strip the volatile species from solution,
as described above. However,
in a series of papers, Brindle and others
showed that low acid concentrations
resulted in better signals from
hydride-forming elements, but they
relied on a frit-based separator with
argon flowing through it to generate
small bubbles, thereby substituting
argon for the chemically generated
hydrogen to strip the volatile analytes
from solution.12 The importance
of gas–liquid separator design on performance
was further illustrated in
a paper by Brindle and Zheng, who
compared a variety of commercial and
purpose-built gas–liquid
separators
for the determination of mercury by
cold-vapor generation.13 The upshot
was that the two designs that performed
best were small dead-volume
systems, designed for applications to
flow-injection systems.
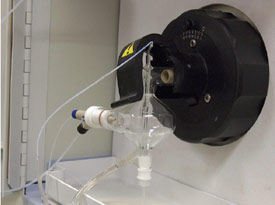
Figure 1 - Multimode Sample Introduction System.
In 2002, McLaughlin and Brindle
published a paper that presented a
radically new design for the combined
nebulizer/gas–liquid separator.14 The Multimode Sample Introduction
System (MSIS) is based on
a cyclonic spray chamber that has
been modified by the addition of
vertically opposed tubes in the center
of the chamber (Figure 1). The
device was patented in May 2005.15
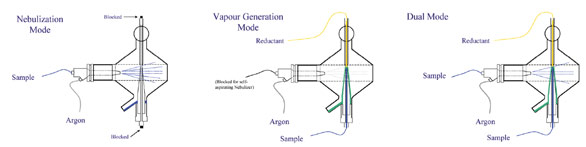
Figure 2 - Modes of operation.
The system is capable of operating
as a conventional spray chamber,
as a gas–liquid separator, or
as both simultaneously. The various
modes of operation are shown
in Figure 2. The MSIS is unique in
its approach to vapor generation in
that it employs nonconfined point
gap mixing of the sample and reductant,
and effective stripping of the
volatile species using thin flowing
film technology. The mixed solution
in this case flows down a conical
ground glass tube to the drain, where
it is continuously pumped to waste.
Gas from the nebulizer strips the
volatile species and delivers them to
the plasma. The sample flow can be
split, delivering part of the solution
for nebulization and the remainder
for hydride generation. These
characteristics virtually eliminate
the possibility of sample cross-talk
that is inherent with reservoir-based
designs discussed above. The MSIS
essentially eliminates signal noise
caused by sputtering that occurs in
manifold systems, where reductant
and sample are mixed in a confined
tube and where generated hydrogen
inevitably results in bubble formation
and pressure increases.
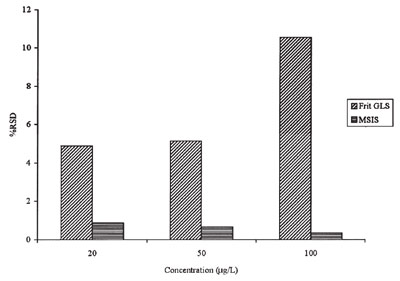
Figure 3 - Comparison of %RSD for the MSIS versus a frit-based gas–liquid separator.
Detection limits for hydride-forming
elements have values of 30 pg
mL–1 for arsenic (this work) and selenium.
The MSIS, unlike frit-based
gas–liquid
separators, delivers performance
similar to a conventional
nebulizer-spray chamber in that the
relative standard deviation (RSD)
decreases with concentration. Frit-based
gas–liquid separators, on
the other hand, show an alarming
increase in RSDs as the concentration
of analyte increases (Figure 3,
adapted from Ref. 14). Furthermore,
operation of the system for extended
periods of time does not lead to the
buildup of droplets in the injector
and, in this respect, the device
acts somewhat like a baffled cyclonic
spray chamber.
Several features of the MSIS have
attracted the interest of a number of
laboratories. The Canadian Association
for Environmental Analytical
Laboratories (CAEAL) has approved
its use for application to the determination of elements in sludges, biosolids,
soils, drinking waters, and wastewaters.
Benefits of the MSIS are 1)
elimination of single-element
technology
(quartz furnace, for example)
and 2) improvement in turnaround
time for sample processing. Data for
two different sample types, run on
an Optima 4300DV (PerkinElmer,
Shelton, CT) operating in axial
viewing mode, have been presented
here. The first sample type was a
water pollution trace metals performance
testing standard (Wibby
Environmental, Golden, CO), and
the second a metals in sewage sludge
certified reference material (CRM)
(Resource Technology Corp., Laramie,
WY).
Results and discussion
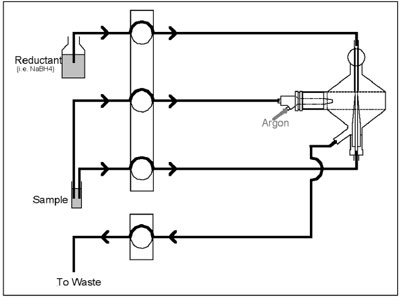
Figure 4 - Dual-mode flow scheme.
The results in Tables 1 and 2 reveal
the quality of data provided by the
MSIS operating in a routine fashion
using dual-mode operation (Figure
4). The Optima 4300 used relatively
short (1–5 sec) instrument-selected
integration times, indicating the
type of performance that could
be expected from an instrument
operating in a typical contract or
municipal laboratory. Results are
almost uniformly of high quality;
only in the sewage sludge standard
reference material does the value
for antimony vary significantly from
the expected value (this discrepancy
has been noted by others, who
report values lower than the certificate),
and the value for barium in
the same sample exhibits unusually
large standard deviation for reasons
that are not clear.
Conclusion
The MSIS is a robust device for the
determination of trace hydride-forming
elements in a production
laboratory. One of the authors
(P. Cheese) has been using it on
a routine basis for more than two
years in the vapor-generation
mode for the determination of As,
Sb, and Se, where it has delivered
satisfactory results in conformity
with CAEAL requirements.
For these elements, the required
method detection limits could not be achieved using standard nebulization.
Dual mode is not used
in the particular case of the environmental
laboratory since boron
must be reported in samples. The
MSIS, coupled with the ICP emission
instrumentation, has enabled
the laboratory to meet turnaround
time requirements, illustrating
how the MSIS delivers efficiency,
as well as accuracy, for the routine
environmental laboratory.
References
- Browner, R.F.; Boorn, A.W. Anal.
Chem. 1984, 56, 787A–98A.
- Sharp, B.L. J.Anal. Atom. Spectrom.
1988, 3, 613–52.
- Sharp, B.L. J.Anal. Atom. Spectrom.1988, 3, 8753–886.
- Nakahara, T.Prog. Anal. Atom. Spectrosc.1983, 6, 163–223.
- Le, X.C.; Cullen, W.R.; Reimer, K.J.;
Brindle, I.D. Anal. Chim. Acta 1992,
258, 307–15.
- Rigby, C.; Brindle, I.D. J.Anal. Atom.
Spectrom. 1999, 14, 253–8.
- Brindle, I.D.; Zheng,S. Spectrochim.
Acta, Part B1996, 51, 1777–80.
- Thompson, M.; Pahlavanpour, B.; Walton,
S.J. Analyst1978, 103, 568–79.
- Wolnik, K.A.; Fricke, F.L.; Hahn,
M.H.; Caruso, J.A. Anal. Chem.
1981, 53, 1030–4.
- Huang, B.; Zhang, Z.; Zeng, X. Spectrochim.
Acta, Part B 1987, 42, 129–37.
- Fengzhou, Q.; Guoying, G.; Chun, X.
Appl. Spectrosc.1991, 45, 287–92.
- Chen, H.; Brindle, I.D.; Le, X.C.
Anal. Chem.1992, 64, 667–72 and
references cited.
- Brindle, I.D.; Zheng, S. Spectrochim.
Acta, Part B 1996, 51, 1777–80.
- McLaughlin, R.L.J.; Brindle, I.D.
J.Anal. Atom. Spectrom.2002, 17,
1540–8.
- McLaughlin, R.L.J.; Brindle, I.D. Multimode
Sample Introduction System.
U.S. patent. Patent no. 6,891,605;
May 10, 2005.
Mr. McLaughlin, Ms. Ding, Mr. Ptolemy,
Ms. Conn, and Dr. Brindle are with the
Chemistry Department, Brock University,
St. Catharines, Ontario L2S 3A1, Canada;
tel.: 905-688-5550; fax: 905-682-9020;
e-mail: [email protected]. Ms. Cheese
is with the Niagara Regional Environment
Laboratory, The Regional Municipality of
Niagara, Thorold, Ontario, Canada.