Analytical laboratories are increasingly
challenged to improve data quality and
increase sample throughput. Exploration
of new ways to meet these requirements
has generated considerable interest in liquid chromatography systems utilizing
sub-two-micron (STM/<2 μm) column
technology. Providers of these instruments
claim reduced run times,
improved resolution, as well as other performance
enhancements.
Because questions have been raised concerning
the accuracy and specificity of some
of these claims, there has been a need for
objective criteria by which to gauge performance
of the various STM-capable LC
instruments. In the article in Ref. 1, authors
from a large pharmaceutical company outline
what they consider to be levels of performance
indicative of the potential of
STM technology. Their criteria include:
- Demonstrating instrumental capability for generating high LC flow rates for faster separations while minimizing increases in backpressure
- Utilizing elevated temperature to reduce separation times
- Accommodating all HPLC and STM-LC operational modes on a
single instrument and facilitating use of HPLC methods without the need for revalidation - Achieving separation efficiencies close to theoretical predictions
- Demonstrating the high-speed STM capability without compromising precision or sensitivity.
To these can be added:
- Maximizing sample throughput by minimizing cycle times
- Ensuring ease of use
- Providing robust system and data security
- Minimizing downtime through proactive diagnostic monitoring and maintenance.
Defining terms
Rapid Resolution liquid chromatography (RRLC, Agilent Technologies, Palo
Alto, CA), also known as ultra high pressure chromatography (U-HPLC) or
ultrafast LC (UFLC), are the terms used
to designate LC applications employing
STM columns with 1.0–4.6 mm i.d.
Pump pressure
LC run times are primarily a function
of flow rate. With 3.5-μm or 5.0-μm
column packing, increases in linear
velocity beyond approximately 1.0
mL/min are accompanied by decreases
in column efficiency, which negatively
affects resolution. At flow rates
of about 2.0 mL/min, the decrease in
column efficiency for 3.5-μm and 5.0-μm particles is roughly 15% and 40%,
respectively. At a flow rate of 5.0
mL/min, the corresponding losses are
40% and 65%, respectively. This is
not the case for STM columns, for
which the efficiency remains fairly
constant over the same flow rate range
(Figure 1). As a result, STM columns
make it possible to shorten run times
considerably without compromising
resolution. (A 5–10 fold run time
decrease is typical, even without increasing temperature.) Sustaining
faster linear flow requires adequate
pumping capacity.
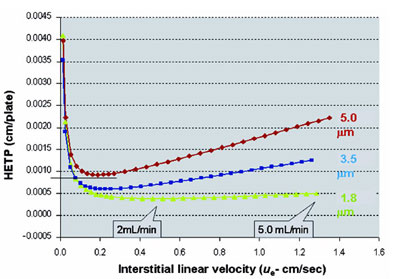
Figure 1 - Column efficiency at increasing flow rate for conventional HPLC vs STM-LC
columns. Unlike columns with 5-μm or 3.5-μm packing, STM-packed columns are efficient at high
linear flows and realize significant gains in analysis speed, typically 5–10× without changing temperature.
The STM column used here is a Zorbax 1.8-μm RRHT (Agilent Technologies) with 4.6
mm i.d. Experiments were performed on an Agilent 1100 series binary LC system.
At a given pumping pressure, a
decrease in packing particle size will
cause a corresponding drop in flow
rate. To overcome this effect, additional
increases in pumping pressure
are needed. Moreover, as linear speeds
increase, more stringent control of
flow rate variations and other deviations
in chromatographic dynamics
are necessary to maintain acceptable
precision. These conditions can be
met by a binary pump capable of producing
pressures up to 600 bar and
flow rates up to 5 mL/min. Perturbations
to constant flow are held within
narrow limits by the pump’s electronic
dampening control system. The result
is precisely reproducible chromatographic
performance (Figure 2) as well
as lower baseline noise.
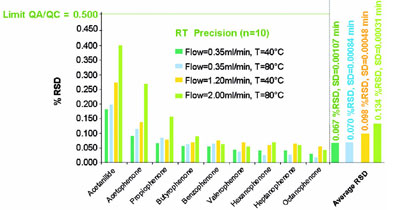
Figure 2 - Maintaining precision despite significant changes in flow rate and temperature. The
1200 series RRLC is equipped with electronic dampening of the system pump piston to minimize flow
rate fluctuations and electronic controls that hold temperature constant to within ±0.05 °C. These
system constraints also lower pump and heater contributions to baseline noise.
While brute pumping force is required
to generate the pressures needed for
faster chromatographic flow rates,
improvements in pumping efficiency
need to be considered as well. Optimizing
the instrument configuration to
minimize its contribution to the overall
backpressure enables greater utilization
of the pump’s capacity for generating
faster flows. Reducing the load on the pump also contributes to longer system
and component life and lowers both
maintenance downtime and operating
costs. To that end, an RRLC system
incorporates additional pressure reducing
characteristics such as:
- More efficient packing of the column and the use of STM columns with particle size distributions designed for pressure reduction (Zorbax 1.8-μm RRHT columns)
- Utilizing system-connecting capillaries with internal diameters and lengths that produce lower backpressure without increasing peak dispersion
- Autosampler valving and electronic controls designed for minimal injection pressure spikes.
The combination of higher pumping
capacity and system-engineered pressure
lowering enables an RRLC system
to operate efficiently over a flow
rate range of 0.05–5 mL/min, giving
linear flow rates of up to 16 mm/sec at
absolute flow rates of up to 2.4
mL/min (5 mL/min) on 2.1-mm (3-mm) i.d. RRHT columns.
Temperature
Because of its inverse relationship to
mobile phase viscosity, temperature is
another parameter that can be manipulated
to increase flow rate. (This may
not apply to non-Newtonian fluids
such as polymers and substances that
form immobilized bridged networks
such as gels.) This is especially the
case for important chromatographic
solvents such as water and alcohols
that exhibit strong intermolecularly
bonded networks that are considerably
weakened, with commensurate reduction in viscosity as the temperature
is increased.
The equation t/N ∝ η, which
describes the relationship between
temperature and viscosity, merits further
attention (t = temperature, N =
theoretical plate number, and η is viscosity).
Rearranging the terms t/η∝N
yields an expression indicating that
column efficiency increases rapidly
with increasing temperature since an
increase in t is accompanied by a
decrease in η. Figure 3 illustrates the
run time reductions gained by the use
of an STM packing in a narrow-bore/short-column format and the
additional reductions realized by running
this setup at elevated temperature.
Note that the decreases in run
times are achieved with resolution
comparable to HPLC. In certain
applications, such as LC-electrospray ionization (ESI)-MS, elevating the
temperature can also improve sensitivity
by increasing the efficiency of
nebulization and desolvation in the
electrospray ionization process.
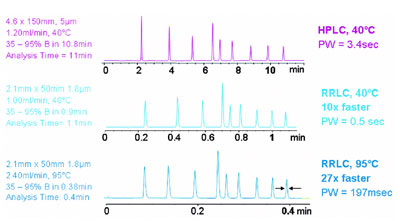
Figure 3 - Maximizing run time reduction. Performing STM-LC in a short, narrow-bore column
at modestly elevated temperature achieves a tenfold decrease in run times on the 1200 series RRLC.
Using the same chromatographic configuration and elevating the temperature results in a 27-fold
increase in analytical speed compared with HPLC performed on a standard-bore column with a standard
particle size packing. STM-LC/temperature gains in analytical speed do not come at the expense
of resolution, sensitivity, or precision, which are comparable to the corresponding HPLC method.
High-temperature LC introduces a
set of constraints that must be properly
managed to avoid potentially
counterproductive effects. First and
foremost, the column packing
chemistry must be thermally stable.
Performance specifications for Zorbax
Stablebond C18 columns used
in the experiments presented here
demonstrate long-term stable operation
at temperatures up to 90 °C
and beyond. The LC column temperature
can be rapidly elevated by
means of a thermostated column
compartment equipped with Peltier-heating
technology. A low-volume
heat exchanger is employed to minimize
peak broadening on narrow-bore
columns.
A second Peltier-controlled heat
exchanger incorporated postcolumn
forms a feedback loop with the flow
cell temperature sensor, ensuring optimal
thermostating of the eluent and
the elimination of temperature-induced
noise in the detector. Low-noise
electronics complement the flow
cells that have been redesigned to
maximize sensitivity. Postcolumn cooling
coupled with fast run times also
significantly reduce the probability of
degradation by limiting analyte residence
time at elevated temperature.
Accommodating conventional HPLC and RRLC operational modes
Instrument flexibility is an especially
important attribute in a period of significant
technology transition. The
ability to accept both narrow- and
standard-bore columns eliminates the
need for separate HPLC and RRLC
instruments. Users can continue to
run current HPLC methods, maintain
the existing work flow, and meet regulatory
requirements while simultaneously
making the transition to RRLC.
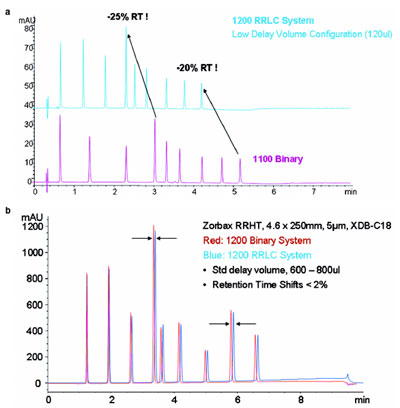
Figure 4 - Selectable delay volume avoids retention time mismatch when migrating methods from
HPLC to RRLC. RT shifts of up to 25% can occur when (a) an inappropriately low delay volume is
used for running RRLC on standard-bore columns. The RT discrepancy limits compatibility between
HPLC and RRLC applications and confounds peak tracking, requiring method revalidation. Selection
of the appropriate 600–800 μL standard delay volume (b) for both RRLC and conventional HPLC
methods run on standard-bore columns aligns run times, eliminating the need for method revalidation.
The lower 120-μL delay volume is reserved for narrow-bore column LC and LC-MS applications.
Adapting standard-bore column
methods to narrow-bore columns
ordinarily results in significant
alterations in retention time.
(Shifts as large as 30% have been
documented.) This large displacement
creates considerable uncertainty
with respect to peak identification, necessitating method
revalidation (Figure 4a). One way
to overcome this problem is to
employ selectable delay volumes: 600–800 μL for standard-bore
columns and 120 μL for narrow-bore
columns. Using the standard
delay volume eliminates the
HPLC-to-RRLC retention time
displacement, which is typically
held to <2%. As a result, existing
HPLC methods can be executed
without revalidation, and a
method changeover from HPLC to
RRLC often requires as little as 5
min (Figure 4b).
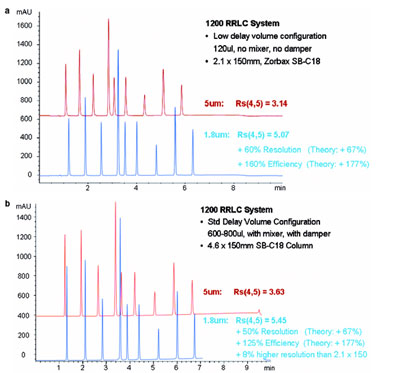
Figure
5 - Near theoretical separation efficiencies for STM-LC in both a)
narrow-bore and
b) standard-bore columns. 1200 RRLC performance in standard-bore columns
shows no sacrifice in
chromatographic efficiency as a result of a thermal mismatch between
mobile phase and column. (Such a
thermal mismatch would cause band broadening due to temperature
gradients across the column radius.)
The data shown here demonstrate nearly comparable column efficiency and
resolution, approaching theoretical
values for both standard- and narrow-bore STM columns.
Apparatus/experimental conditions—Test sample: set of nine compounds,
100 ng/μL each, dissolved in acetonitrile (ACN). 1)
Acetanilide, 2) acetophenone, 3) propiophenone, 4) butyrophenone, 5)
benzophenone, 6) valerophenone,
7) hexanophenone, 8) heptanophenone, 9) octanophenone. Instrument: 1200
Series RRLC with
binary SL pump, high-performance Autosampler SL, thermostated column
compartment, diode array detector SL (13-μL cell, 20-Hz data acquisition rate slit: 4 nm, signal: 245 nm). Columns: 4.6 and 2.1
× 150 mm SB-C18, 5 μm and 1.8 μm. Solvent A: H2O + 0.1% trifluoracetic acid (TFA). Solvent B:
ACN + 0.095% TFA. Gradient: 50–95% B in 7 min, hold over 1 min. Stop time: 10 min. Flow rate:
1.5 mL/min. Injection volume: 3 μL. Wash: 5 sec. Temperature: 50 °C.
Achieving separation efficiencies close to theoretical prediction
Run time reduction is not the only
objective of STM technology. The
term “rapid resolution” indicates
that improved separation efficiency
is also an important objective.
Figure 5a and b compare runs on 5-μm and 1.8-μm Zorbax 2.1 × 150 mm
and 4.6 × 150 SB-C18 columns,
demonstrating efficiency gains close
to theoretical prediction on both
narrow- and standard-bore columns.
Figure 5 also demonstrates that the
highest absolute resolution is
achieved on 4.6-mm columns, which
show an inherently higher efficiency
than 2.1-mm columns.
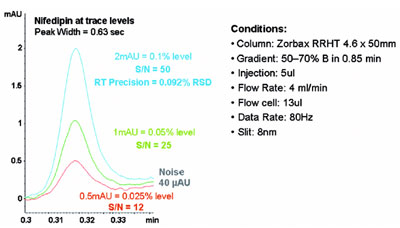
Figure 6 - Accurate impurity quantitation with rapid run times. Under ultrafast LC conditions,
the 1200 Series RRLC equipped with an Agilent DAD/MWD SL allows accurate quantitation of
impurities and side products at levels smaller than 0.05% of the main compound(s).
As retention times decrease, peaks
narrow (0.2–1 sec widths are typical)
and detection sampling rates must be
increased to preserve resolution. Use
of a low-noise diode array detector
(DAD) that can sample up to 80 Hz
makes it possible to routinely
achieve a S/N >10 at 0.5 mAU or
0.03% level, without dispersing
peaks by inappropriate low sampling
rates. This is in excess of the sensitivity
required for quantification of
pharmaceutical side products below
the critical 0.05% of the main compound
(Figure 6). Table 1 provides
further insight into the tradeoff
between data sampling rate, data
quality, and sensitivity. It shows that
a properly designed LC system can
provide significant flexibility to
adjust these parameters for various
application requirements.
Fast run times: No assurance of high analytical throughput
Minimizing turnaround time is vital if
the savings from fast run times are to be
translated into high-volume sample
throughput. One approach to reducing
cycle times for high-throughput operations
is called alternating column regeneration
(ACR). This is a tandem, dual-column
configuration in which one
column is flushed and equilibrated while
the other performs a chromatographic
separation. ACR can reduce cycle times
by as much as 50% and produce throughputs
of more than 2000 samples per day.
ACR is enabled by means of a two-position/10-port valve system that
controls the timing and fluid paths
required for each phase of the chromatography
and/or column regeneration
(Figure 7). Because column flush and regeneration intervals are typically
shorter than analysis times, additional
means may be employed to further
shrink cycle times. Examples
include using an automatic delay volume
reduction that bypasses the sampling
assembly directly after the sample
has been injected and before the
gradient is introduced onto the
column (6 μL on the 1200 highperformance Autosampler SL) and
the ability to prepare the next injection
while the analysis takes place in
bypass mode, which is typically
referred to as overlapped injections.
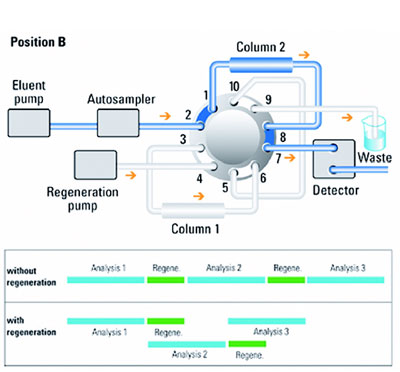
Figure 7 - ACR-enabling two-position/10-port valve system.
ACR saves up to 50% of cycle time by utilizing parallel
column wash and regeneration, overlapped injections, and
accelerated sampling operations. Throughputs of more than
2000 samples/day can be achieved.
Reducing the intervals for operations
such as sampling and column
regeneration requires additional
measures to ensure that injections
remain precise and that carryover
between successive runs is minimized.
In certain instances, both
objectives can be facilitated with
the same technology solution. For
example, directing solvent flow
through the sampling assembly
(needle, loop, and metering device)
ensures continual flushing of the
internal surfaces. Simultaneous
external washing of the needle
helps prevent contamination of the
needle seat. Automatic additional
valve switches at different programmable
or automatic time points
provide the means for further flushing
persistent residues from the system.
High-throughput operations
are also beneficially supported by
the use of large solvent reservoirs
that permit unattended operation
for extended periods.
Control, compliance, usability, and servicing issues
System design that incorporates robust
user support helps to maximize performance
and ease of use while minimizing
downtime and operating costs. Especially
useful are instrument controller
enhancements such as highly readable
displays and the elimination of time-wasting
routines such as those requiring
system restarts whenever a configuration
is changed. Advances in electronics
make a faster software response possible,
and the extension of centralized control
to a larger universe of instrument components.
Controllers that incorporate
these innovations enable more rapid and
comprehensive data management (analysis,
retrieval, and review) and improved
system navigation.
LC instruments at the cutting edge now
offer a multiplicity of interactive help
functions that gauge performance;
proactively indicate when maintenance
is needed; and provide straightforward,
user-guided diagnostics. These routines
increase the efficiency with which the
instrument asset is managed by implementing
timely parts replacement and
rapid deployment of the requisite servicing
resource, when necessary.
A number of measures are increasingly
seen as intrinsic to secure and compliant
instrument and laboratory operations.
These include data loss protection in
the event of a communication interrupt
and electronic tags that unambiguously
track system parameters. Data and system
security are addressed through multiple
layers of protection, including
Internet firewalls, user control of communications,
end-to-end data encryption,
and compliance with network
security. Access is typically password
protected and can be ranked according
to a system of privileges.
Conclusion
The data presented here demonstrate
unequivocally that the 1200 series
RRLC meets the range of criteria for
delivering the full performance
potential of STM
technology as well as supporting
conventional HPLC.
It also shows that the system
provides an easy and secure
transition between the two
technologies. This flexibility
derives from the instrument
platform design that comprises
a matrix of functional
modules that can be configured
and reconfigured to
meet a diversity of analytical
LC applications. The various
configurations constitute a
continuum or glide path
across performance regimes
that address current and
anticipate future needs.
Users entering the 1200 series continuum with an 1100 series
emulation or by upgrading an 1100 series
instrument with 1200 series modules are
well positioned to reconfigure their system
for RRLC operations. Naturally, one
can start out with a fully equipped 1200
RRLC system configuration.
Reference
- Kofman, J.; Zhao, Y.; Maloney, T.; Baumgartner, T.; Bujalski, R. Ultra-high performance liquid chromatography: hope or hype? http://www.chem.agilent.com/Library/articlereprints/Public/5989-5169EN-lo.pdf.
Dr. Frank is Application Chemist, Dr.
Gratzfeld-Huesgen is Application Chemist, and
Dr. Schuette is Program Manager 1200 Series
Rapid Resolution System, Agilent Technologies,
Hewlett-Packard-Str. 8, 76337 Waldbronn,
Germany; tel.: +49 7243 602820, ext.
510; e-mail: [email protected].