Analysis of cells and cellular processes in biological
and medical research requires systems for imaging
as well as tools for manipulating and dissecting cells
and subcellular structures. Femtosecond laser technology
is applicable for both. Imaging using femtosecond lasers is known as multiphoton microscopy,
and is already well established. Near-infrared radiation
is also very well suited for gentle manipulation
of living cells and subcellular structures. Different
research groups are engaged in using ultrashort laser
pulses for nanodissection; however, only a few commercial
systems are available, including the system
described here. The CellSurgeon laser nanodissection
system (Rowiak GmbH, Hanover, Germany)
has been developed in collaboration with the Medical
Technology Department of the Laser Centre
Hanover (Germany). It can be used to manipulate
cell dynamics, deactivate cell organelles, or
influence cellular processes such as metabolism and
apoptosis. An overview of the technology follows,
including information on its potential for two different
applications.
Principle and advantages
The main component of the CellSurgeon system
is a tunable NIR femtosecond laser coupled to
a microscope objective. Depending on the configuration,
the pulse repetition rate varies between
several kilohertz and 90 MHz. The laser is moved
by software-controlled scan mirrors, enabling various
cutting geometries such as single point and
lines as well as predefined or freehand shapes. A
three-axis piezo-driven microscope stage permits
positioning of samples with a resolution of 20 nm
in each direction.
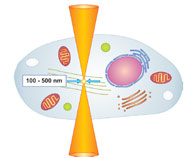
Figure 1 - Nanodissection is induced within the focal volume of a
tightly focused femtosecond laser.
Nanodissection with ultrashort pulses is based on
nonlinear absorption effects, which occurs when
high laser intensities are confined to a very small
volume (Figure 1). This is realized by tight focusing
of the laser beam using high-numerical-aperture
objectives. If the energy per laser pulse increases above a certain threshold, the photon density
in the focal volume rises high enough
to induce multiphoton
absorption and avalanche
ionization. These processes lead
to a very high concentration of free electrons
within the focal region, resulting in
plasma-mediated ablation of material.1
Depending on the numerical aperture of
the microscope objective and the laser
pulse energy, the lateral extent of the
focal volume and thus the interaction
with the sample can be limited to less
than 1 μm. That allows the manipulation
or dissection of single-cell organelles,
which have a typical size of a few
micrometers. For precise dissection, the
energy has to be as low as possible, while
the numerical aperture of the objective has to be
high. One key advantage of femtosecond lasers
is that at a pulse duration of 140 fsec, only a few
nanojoules of energy are necessary for dissection.
In contrast, dissection with UV laser systems
requires energies that are at least two or
three orders of magnitude higher. The low laser
energies allow very precise cuts with minimum
dimensions of approx. 100 nm and cause negligible
collateral damage, reducing the risk of
injuring or killing the cell.
Applications
The laser nanodissection system is especially suited
for applications in medical and biological research.
Manipulation and dissection of cells or subcellular
structures can be useful for investigations of cellular
processes such as metabolism, apoptosis, or cell
dynamics. An understanding of cellular and subcellular
mechanisms is important for the development
of drugs and stem cell or gene-based therapies against
diseases such as cancer or Alzheimer’s and Parkinson’s
disease.
Two examples of experiments are given in
order to help the reader visualize potential
applications. All experiments were conducted
at the Laser Centre
Hanover by the Biophotonics
Research Group (part of the Biomedical
Optics Dept. of the Laser Centre Hanover).
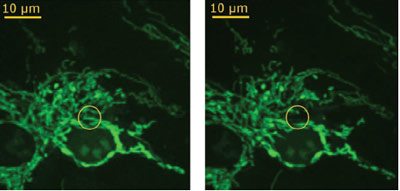
Figure 2 - Fluorescence image of endothelial cells. Left: cells before manipulation;
single mitochondrion to be ablated is marked by yellow circle. Right:
cells after manipulation; the ablation was realized at a pulse energy of 1 nJ,
and the ablated mitochondrion is not visible. (Figure courtesy of J. Baumgart,
Laser Centre Hanover; reproduced with permission from Ref. 2.)
The first experiment demonstrates the deactivation
of specific cell structures. In this
case, mitochondria in living endothelial
cells were disrupted to study the induction
of cell death. The mitochondria disruption
was realized at pulse energies between 0.7
and 1.0 nJ and with a cutting speed of 14
mm/sec at a pulse repetition rate of 90 MHz. Fluorescence imaging was used to investigate
changes of the mitochondria arrangement.
For this microscopic examination, the
cells were stained with MitoTracker Orange
(Molecular Probes, Eugene, OR). Figure 2
shows an example of a treated endothelial
cell before and after the disruption of a single
mitochondrion. To receive a first impression of the viability after the laser manipulation, the
treated cells were observed over a period of 1
hr with both fluorescence imaging and brightfield
microscopy imaging. Usually, apoptotic or
necrotic cells change their volume, which can be
seen under the brightfield microscope. In none of
the studies did the ablation of a single mitochondrion
lead to the induction of cell death during
the observation time. Certainly, further study
with longer observation time is required to draw
a solid conclusion. First results, however, are
promising that cell organelles can selectively be
ablated by laser nanodissection without inducing
further cell damage or even cell death.2
Another possible application of laser nanodissection
is the optical perforation of cell membranes
to enable the transfer of genes into living
cells. By focusing the laser beam for 20–40 msec
on the membrane, transient pores are created so
that fluorescent molecules or nucleic acids can
enter into the cell. Conventional techniques to
permeabilize the cell membranes involve the use
of viral vectors, chemical carriers, or electroporation.
However, the application of these techniques
for primary cells or stem cells, especially
with limited populations, can be critical or impossible
due to low success rate or severe side effects.
For these cell types, optical membrane perforation
provides an interesting alternative because it is a
very gentle and precise method, allowing targeted
perforation of single cells.
DNA transfection by laser nanodissection requires
a detailed examination of the laser parameters for
the cell type used. The motivation for the following
set of experiments was to find out the optimum
laser parameters for membrane perforation in order
to induce an uptake of fluorescent molecules, more
precisely propidium iodide (PI). This fluorochrome
is commonly used to stain DNA and to differentiate
necrotic, apoptotic, and normal cells.
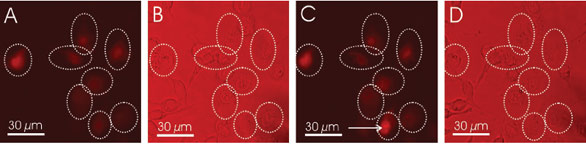
Figure 3 - Opto-perforated granulosa cells in the presence of PI. a) Fluorescence image of granulosa cells during opto-perforation; the
treated cells are highlighted by the dashed circles. The cells were treated at a pulse energy of 0.9 nJ and an irradiation time of 40 msec.
All manipulated cells are fluorescent. b) Brightfield image of the same cells. c) Fluorescence image of the cells after 90-min incubation
in PBS. The cells were restained with PI to verify the viability. The cell pointed out by the arrow is representative for a cell whose membrane
is damaged and therefore still permeable for the fluorophore. d) Brightfield image after the incubation time. (Figure courtesy of J.
Baumgart, Laser Centre Hanover; reproduced with permission from Ref. 3.)
The aim of the experiments was to analyze the
relationship between pulse energy, irradiation
time, repetition rate, and transfection efficacy
as well as the viability of the cells. For the laser
manipulation, a coverslip with GFSH-R17 granulosa cells of rat was transferred into a perfusion chamber containing 0.5 mL
of phosphate-buffered saline (PBS) and 1.5 µm PI. By focusing the laser with
a 0.8-NA NIR water immersion objective (Achroplan, Carl Zeiss AG, Jena,
Germany), the focus had a theoretical size of approx. 600 nm. The cells were
perforated at a central wavelength of 800 nm and a repetition rate of 90 MHz.
Perforation of the granulosa cells was realized at pulse energies in the range
of 0.7–1.1 nJ at irradiation times between 30 and 60 msec. Every parameter
combination was tested with 40–60 cells. After manipulation, the cells were
observed by fluorescence microscopy to verify the induced fluorescence of the
PI, and then washed with PBS and incubated in PBS for 90 min. Finally, the
viability of the treated cells was controlled by relabeling with PI and comparing
the fluorescence intensity before and after restaining. Cells with an intact
physiologic structure are impermeable for PI molecules. They show only very
low fluorescence, emitted by fluorophores uptaken directly after the optoperforation.
In contrast, the membrane of damaged cells becomes completely
permeable for fluorophores and can be identified by its higher fluorescence
intensities (Figure 3).
The efficiency of dye uptake increased with the pulse energy and the irradiation
time, while the viability observed 90 min after the treatment decreased.
In detail, the viability varied between 20 and 90%
and the efficiency between 10 and 90%. The best
compromise between efficiency and viability was
found at a pulse energy of 0.9 nJ and an irradiation
time of 40 msec. These parameters led to an efficiency
of 70% and a viability of 80%. Certainly, this
is a satisfactory result.
As a proof of principle, these optimized parameters
were successfully used to transfect canine mammary
cells (MTH53a) with a vector coding for a GFPHMGB1
fusion protein. The transfected cells were
observed up to 48 hr after the treatment and the cells
did not show any signs of apoptosis or necrosis.3 These
first results display the potential of laser nanodissection
for cell transfection.
Summary
The CellSurgeon is a versatile tool that can open new horizons for scientists who are
primarily interested in the investigation of cells and cellular processes. Nanodissection
with femtosecond lasers can help solve various problems in cell and molecular
biology as well as in medicine or pharmacy. Cells, their organelles, and structures can
be selected with high accuracy and high reproducibility. The technology allows targeted
manipulation of cell parts without damaging others. This considerably improves
the success rate of cell manipulation and thus the efficiency of laboratory work.
References
- Vogel, A.; Noack, J.; Huettman, G.; Paltauf, G. Mechanisms of femtosecond laser nanosurgery
of cells and tissues. Appl. Phys. B 2005, 81(8), 1015–47.
- Heisterkamp, A.; Baumgart, J.; Maxwell, I.Z.; Ngezahayo,
A.; Mazur, E.; Lubatschowski,
H. Fs-Laser Scissors for Photobleaching, Ablation in Fixed Samples and Living Cells,
and Studies of Cell Mechanics. In Laser Manipulation of Cells and Tissues; Elsevier Inc.:
New York, NY, 2007.
- Baumgart, J.; Bintig, W.; Ngezahayo, A.; Willenbrock, S.; Murua Escobar, H.; Ertmer, W.;
Lubatschowski, H.; Heisterkamp, A. Femtosecond laser based opto-perforation
of living
GFSHR-17 and MTH53a cells. Optics Express 2008, 16, 3021–31. www.opticsinfobase. org/abstract.cfm?URI=oe-16-5-3021.
Ms. Menne is with Rowiak GmbH, Garbsener Landstrasse 10, D-30419 Hanover, Germany;
tel.: +49 511 277 2952; fax: +49 511 277 2959; e-mail: [email protected].